madman
Super Moderator
Role of sex hormone‑binding globulin in the free hormone hypothesis and the relevance of free testosterone in androgen physiology (2022)
N. Narinx · K. David1, · J. Walravens · P. Vermeersch · F. Claessens · T. Fiers · B. Lapauw · L. Antonio, · D. Vanderschueren
Keep in mind this is Fier's camp (cFTV).
Nothing new that those in the know were already well aware of!
You heard it here first the one and only Nelson's ExcelMale!
Abstract
According to the free hormone hypothesis, the biological activity of a certain hormone is best reflected by free rather than total hormone concentrations. A crucial element in this theory is the presence of binding proteins, which function as gatekeepers for steroid action. For testosterone, tissue exposure is governed by a delicate equilibrium between free and total testosterone which is determined through interaction with the binding proteins sex hormone-binding globulin and albumin. Ageing, genetics and various pathological conditions influence this equilibrium, hereby possibly modulating hormonal exposure to the target tissues. Despite the ongoing controversy on the subject, strong evidence from recent in vitro, in vivo, and human experiments emphasizes the relevance of free testosterone. Currently, however, clinical possibilities for free hormone diagnostics are limited. Direct immunoassays are inaccurate, while gold standard liquid chromatography with tandem mass spectrometry (LC-MS/MS) coupled equilibrium dialysis is not available for clinical routine. Calculation models for free testosterone, despite intrinsic limitations, provide a suitable alternative, of which the Vermeulen calculator is currently the preferred method. Calculated free testosterone is indeed associated with bone health, frailty, and other clinical endpoints. Moreover, the added value of free testosterone in the clinical diagnosis of male hypogonadism is clearly evident. In suspected hypogonadal men in whom borderline low total testosterone and/or altered sex hormone-binding globulin levels are detected, the determination of free testosterone avoids under- and overdiagnosis, facilitating adequate prescription of hormonal replacement therapy. As such, free testosterone should be integrated as a standard biochemical parameter, on top of total testosterone, in the diagnostic workflow of male hypogonadism.
Introduction
Steroid hormones are biological signal transducers that are vital in coordinating diverse developmental and physiological processes. From fetal life into adulthood, steroid hormones regulate different important functional axes in the human body. Physiologically, steroid hormones are divided into three categories: sex steroids (androgens, estrogens, and progestogens), corticoids (mineralocorticoids and glucocorticoids), and vitamin D [1]. While all of these steroid hormones serve a different physiological purpose, their steroidogenesis all starts with the same molecule, cholesterol. Hence, structurally, each type of steroid hormone is based on the classic cyclopentanophenanthrene four-ring structure, or a derivative [2]. Because of their lipophilic properties, the transportation of steroid hormones to target organs and tissues via the bloodstream is mediated through binding proteins (BPs). In fact, the majority of steroid hormone molecules adhere to these BPs, leaving only a small fraction that circulates freely in human plasma [3]. The free hormone hypothesis delivers a rational approach to the biological activity of these different fractions, thereby redefining hormonal exposure in terms of free steroid hormones.
Notwithstanding emerging evidence on the subject, the free hormone hypothesis remains somewhat controversial [4]. Multiple elegant manuscripts reviewing free fraction hormones have been published in the last decade, each one conveying a slightly different message to its readers [3–5]. Accordingly, the debate around free testosterone (T), and its potential use in clinical settings, caused the formation of two movements. While the majority of societies on endocrinology have implemented free T into their guidelines on male hypogonadism, the Australian Endocrine Society does not recommend its usage for clinical decision-making [6, 7]. The purpose of this review is to update current views on the free hormone hypothesis using recent knowledge. In this review, we will highlight recent results from important in vitro, in vivo, and human studies from our group and others, thereby refocusing the use of free T and driving its implementation into the clinical context. As such, we will demonstrate the need for free T in both male and female patient populations, for example in the diagnosis of male hypogonadism and polycystic ovarian syndrome (PCOS). Furthermore, we will discuss the methodology for measured and calculated free T in great detail, including recent data showing that the Vermeulen formula deserves clinical recommendation as the free T calculator of choice. After highlighting the biological relevance and added diagnostic value of free T in the clinical setting, we will conclude with a research agenda giving an overview of open questions and obstacles yet to be cleared.
Steroid hormone‑binding proteins: gatekeepers of hormonal exposure
An essential factor to be considered in the free hormone hypothesis is the contribution of plasma BPs. In human steroid physiology, several BPs have been identified. Not only do these BPs provide the transport of steroid hormones from their production to their target site, but they also contribute considerably to the equilibrium between the free and protein-bound fractions in plasma. As such, BPs function as primary gatekeepers of steroid action [8].
Binding proteins define steroid hormone fractions
Production of steroid hormones occurs at different sites in the human body, delineating a specific process for each hormone. Following biosynthesis, transport to target organs and tissues is facilitated by a group of plasma BPs that are either specific or non-specific carriers. Specific steroid carriers include SHBG which preferentially binds T, dihydrotestosterone (DHT), estradiol, and other sex steroids, vitamin D-binding protein (DBP) for vitamin D and its metabolites, and cortisol-binding globulin (CBG) that preferentially binds cortisol, aldosterone, and progesterone. Additionally, thyroid-binding globulin (TBG) and transthyretin act as specific carriers for thyroid hormones. Next to specific transport, the most abundant human plasma protein, albumin, provides non-specific means of transportation for all previously mentioned components. As a result, three plasma hormone fractions can be defined: the non-protein-bound (free) fraction, the fraction bound to specific BPs (e.g., SHBG), and the albumin-bound fraction (Fig. 1). The free hormone concentration is determined by the level of total hormone, as well as the level of BP and albumin in circulation and the binding affinity of a hormone for its carrier(s), thereby characterizing the equilibrium state between the free and the bound fractions.
SHBG and albumin define a delicate equilibrium in testosterone fractions
The bulk of T transportation is operated by three carriers: SHBG, CBG, and albumin, respectively, representing 44, 4 and 50% of the total T fraction in human male plasma [9]. While the vast majority of circulating T is protein-bound, on average only 2% circulates freely [9]. Human SHBG is a glycoprotein that is mainly produced by the liver. It circulates as a 90–100 kDa homodimeric protein and is able to bind all androgens and estrogens, with the exception of dehydroepiandrosterone sulfate (DHEAS) and androstenedione. Recent studies on UK biobank data indicated that the median plasma SHBG concentration is 36.89 nmol/L in men. In women, median SHBG plasma concentration was valued at 60.34 and 53.56 nmol/L for pre- and postmenopausal age, respectively [10, 11]. This carrier protein is encoded by the SHBG gene, found on the short arm (p12-13) of chromosome 17, consisting of eight exons [12]. Besides expression in the liver, the gene is also transcribed in the brain, uterus, breast, ovary, placenta, prostate, and testis [13]. The binding of sex steroid metabolites is accomplished in a binding pocket of the homodimeric protein, formed through the association of two monomers, stabilized by the bivalent cations zinc and calcium, with each homodimeric protein holding up to two molecules. A serine residue at position 42 in the binding pocket is responsible for the formation of hydrogen bonds with functional groups of T and estradiol [5, 14, 15]. The dynamic relationship between T and SHBG is characterized by their binding affinity, a measure of the strength of interaction between metabolite and carrier. Among steroid hormones, T has the second highest association constant (Ka), valued at 1–2 × 109 M−1 s−1, whereas DHT has the highest Ka for SHBG (2.69 × 109 M−1 s−1) [16]. Therefore, binding between T and SHBG occurs rapidly and is very strong with little tendency for spontaneous dissociation. Binding affinities of SHBG, and all other previously mentioned BPs, have been subject to extensive experimental research, summarized in Table 1 [9, 16].
Albumin, designated as a non-specific transporter of T in the context of the free hormone hypothesis, is the most abundant protein in human plasma. It is a 66.5 kDa protein, circulating at a concentration of 35.0 to 50.0 g/L in both men and women, and is mainly responsible for maintaining colloid osmotic pressure [17]. It also plays a major role in the transportation and homeostasis of diverse ligands (electrolytes, fatty acids, hormones, vitamins, and drugs…) [18]. Synthesis of albumin only occurs in the liver, initiated by transcription of the ALB gene located on chromosome 4 (chr4q13.3) [19]. The binding affinity of T for albumin (Ka of±4× 104 M−1 s −1) is five orders of magnitude smaller and weaker than the binding affinity for SHBG. However, genetic variation in albumin could contribute to altered plasma albumin concentrations and/or binding affinity for T. To date, 71 genetic variants of albumin have been identified. One of these albumin variants, Roma c.1033G > A, has been linked to reduced binding of T [20]. Lower binding affinity for albumin, which has also been demonstrated for all other steroid and thyroid hormones, has raised discussion about the relevance of this albumin-bound fraction. Indeed, the albumin-bound fraction could, because of more probable spontaneous dissociation, potentially also have an effect on biological activity [21, 22]. Hence, the sum of the free and the albumin-bound fraction was determined to constitute the biologically available (or bioavailable) amount in circulation (Fig. 1). More recently, however, the interaction between the albumin-bound and free fraction has become even more complex. The binding dynamics of T to albumin have been generally believed to be in a 1:1 stoichiometric way. However, the structural location of the binding sites in albumin by two-dimensional nuclear magnetic resonance has shown that the carrier protein possesses at least three binding sites for T, each with its own association constant. Moreover, evidence has been provided that these binding sites are allosterically coupled and, importantly, shared with free fatty acids and some commonly used drugs, such as naproxen, warfarin, and exogenous glucocorticoids [23, 24]. Binding site competition and allosteric coupling can in certain conditions, especially postprandial and in circumstances of heightened levels of fatty acids and drug usage, result in displacement of T from its binding site thereby potentially affecting T bioavailability and/or metabolism [25, 26].
The link between binding proteins and the free hormone hypothesis
All BPs, whether specific or non-specific, together control tissue availability and metabolic clearance rate of a given hormone through regulation of the free hormone fraction. In the free hormone hypothesis, it is mostly this free fraction, and by extension the free hormone concentration, that accounts for the biological activity of hormone action
Unraveling the free hormone hypothesis: a model‑based approach
The free hormone hypothesis asserts that the biological activity of a given hormone is affected by the unbound (free) rather than the protein-bound plasma concentration [27]. In 1952, Recant and Riggs observed that patients suffering from nephrotic syndrome were euthyroid, without clinical signs of hypothyroidism, while in contrast, their serum levels of thyroid hormone were far below the normal range. They, therefore, hypothesized the existence of an, at that moment analytically undetectable, unbound fraction exerting biological activity rather than the protein-bound fraction. While suffering from hypoproteinemia and concordant low protein-bound thyroxine, patients would thus still have a sufficient supply of free, unbound, thyroxine [28]. This observation was later formalized by Robbins and Rall in 1957, stating that the concentration of free thyroid hormone in the blood is the main determinant of thyroid hormone action. In accordance, the amount of thyroxine metabolized may also be a function of the level of free thyroxine in serum [29]. In the following years, major advances were made as BPs were characterized and knowledge of a free hormone pool, which is in continuous equilibrium with the protein-bound pool, became well established [30]. Additionally, similar experiments on the sex steroids T and estradiol were conducted by Heyns and De Moor. They succeeded to isolate the free from the protein-bound fraction, thereby enabling them to study the binding kinetics of these sex steroids and their respective BPs [31].
Although many insights were achieved in terms of free serum hormones, it was not until 1989 that a sound mathematical and physiological model for the hypothesis was introduced by Mendel, referring to the hypothesis now generally accepted as the free hormone hypothesis [27]. Despite being broadly dispersed and acknowledged, it is commonly confounded with the assumption that only the non-protein-bound (free) fraction of hormones are able to cross the cell membrane to the intracellular compartment, referred to as the free hormone transport hypothesis [32]. While the free hormone transport hypothesis might be valid, it does not then automatically follow that only the free hormone concentration in the plasma can affect intracellular hormone concentrations and, therefore, exert biological activity. Indeed, this last statement was illustrated by Mendel’s mathematical models [27]. These models characterize the process of cellular steroid hormone uptake through four parameters: plasma flow rate, hormone dissociation rate from its BP, tissue influx rate, and intracellular elimination rate. In each of the proposed models, all representing different tissue types, one of these four factors operates as the rate-limiting factor in the uptake process. This in turn affects the validity of the free hormone hypothesis (Fig. 2). For instance, whenever intracellular elimination is considered the rate-limiting step in the net tissue uptake, the free hormone hypothesis is valid. This implies that intracellular hormone concentration, and, therefore, the biological activity of this hormone, is predominantly determined by its free fraction. The hypothesis is, however, invalid when flow or dissociation from the BP is rate-limiting. In the case of rate-limitation by the influx, the free hormone hypothesis may be either valid (influx via the free hormone pool) or invalid (influx via the protein-bound hormone pool). Providing this knowledge, some general observations can be stated. If a particular hormone exerts an intracellular effect, but its structure is not metabolically altered by the cell, uptake of that hormone is by definition elimination-limited, pleading a valid hypothesis. In contrast, tissues that rapidly metabolize and thus alter the hormonal structure, for instance, metabolic clearance by hepatic tissue, are not elimination-limited pleading against the hypothesis. In summary, these findings highlight that the free hormone hypothesis is not expected to be valid under all possible sets of conditions. A single steroid hormone can be subjected to different models depending on the tissue type [27].
The megalin/cubilin model does not impair the free hormone hypothesis
Although well established by Mendel, various researchers challenging the free hormone hypothesis have tried to demonstrate that the biological activity is mainly exerted by the protein-bound rather than the free fraction. The proposed mechanism for uptake of the hormone–BP complex is through the action of megalin and cubilin, via the process of receptor-mediated endocytosis. Megalin and cubilin are both large, multiligand, endocytic-membrane glycoproteins that are expressed in a wide variety of tissues, including but not limited to the kidneys, brain, endocrine glands, and gonads. Functioning either individually or as a cooperative unit, they are imperative for the endocytic uptake of low molecular weight molecules such as DBP, hormones, enzymes, and lipoproteins [33]. A megalin-knockout mouse model was used to provide evidence for the existence of a megalin-mediated cellular uptake pathway for the internalization of the T-SHBG complex. When megalin was absent, mice would exhibit an impaired testicular descent into the scrotum, delivering evidence that the uptake of the protein-bound fraction is also relevant for intracellular hormone action [34]. This research has, however, been challenged because of multiple flaws in the research design, hence not supporting the “bound steroid” hypothesis [35]. One of the counterarguments stated that mice lacking megalin suffer from severe vitamin D deficiency, accounting for the impaired gonadal development and function, rendering deductions for the T-SHBG complex incorrect [35].
While not applicable to T and SHBG, megalin- and cubilin-mediated endocytosis is, however, relevant in vitamin D metabolism. Being mainly expressed on the luminal brush border membrane of the renal proximal convoluted tubule, megalin and cubilin bind and reabsorb the vitamin D–DBP complex from the glomerular ultrafltrate, hereby preventing uncontrolled loss of vitamin D metabolites in urine. Additionally, megalin-knockout mice as well as patients with diabetes or chronic kidney disease (CKD), in whom renal megalin expression and function are reduced, show extensive loss of both vitamin D metabolites and DBP in the urine leading to a higher risk of vitamin D insufficiency [36, 37]. It is, however, important to state that the kidney is one of the major metabolizer organs in vitamin D biotransformation. Hydroxylation of 25(OH)D to its most active form, 1,25(OH)2D, by CYP27B1 is performed in renal tissue with the supply of 25(OH)D depending on megalin-/cubilin-mediated endocytosis [38]. Mendel’s mathematical model for the free hormone hypothesis describes that metabolizer organs or cells, in this case, cells of the proximal convoluted tubule, have by definition a non-elimination limited uptake and, therefore, are not subject to the free hormone hypothesis. Hence, megalin-/cubilin-mediated uptake of the vitamin D–DBP complex is not a valid counterargument to the free hormone hypothesis [27]. To summarize, while of crucial importance in vitamin D physiology, the action of the megalin and cubilin complex is not applicable to sex steroids and SHBG (Fig. 3).
Core support to the free hormone hypothesis: in vitro and in vivo data
Since its postulation by Mendel in 1989, the understanding of the free hormone hypothesis has advanced significantly. New insights could possibly contribute to the expertise of the daily management of many endocrine disorders. The clinical relevance and utility of this concept are determined by the relationship between the different fractions of the plasma hormone pool and the clinical manifestation of the corresponding affected endocrine axis. Currently, serum total T concentration is still most often used as the primary determinant for the assessment of androgen status in clinical practice. However, the consensus in the discussion on the most clinically useful measurement of sex steroids, total or free concentration, is yet to be reached [39]. In what follows, we present in vitro and in vivo data, delivering arguments in favor of the free hormone hypothesis, emphasizing the importance of SHBG and the relevance of the determination of the non-protein-bound fraction. Hence, this review pitches the measurement of free T as an added value on top of total T.
In vitro
Assessment of in vitro androgen bioactivity was studied by our research group using a stable HEK-293 cell line transfected with a human androgen receptor cDNA construct [40]. Expressing the human androgen receptor, androgen activity is reported through a luciferase-coupled androgen response element (Fig. 4). Using this reporter assay, it was demonstrated that adding recombinant human SHBG to SHBG-deficient serum significantly reduces androgen bioactivity in vitro, hereby confirming the inhibitory effect of SHBG on androgen exposure [41]. In an extension of this first experiment, the impact of genetic variants of SHBG on androgen bioactivity was also investigated in vitro. As mentioned before, SHBG genetic variants affect SHBG and total T concentrations. However, genetic variation in SHBG also alters the binding affinity constant for T and DHT [42]. According to the free hormone hypothesis, it should then follow that an alteration in binding affinity influences the equilibrium between the SHBG-bound and free fraction and thus ultimately impacts androgen activity. Indeed, the usage of genetic SHBG variants in combination with the luciferase androgen reporter assay modifies in vitro androgen bioactivity and reveals increased activity for genetic variants that lower binding affinity [41]. These results are in line with previously conducted in vitro studies that already demonstrated the inhibitory effect of SHBG on T action or uptake [43, 44]. Furthermore, we confirmed these findings in primary cultures of seminal vesicles [45]. In conclusion, these in vitro experiments highlight the importance of SHBG and its inhibitory effect on androgen bioactivity, conveying a major role to the carrier protein and further fortifying the validity of the free hormone hypothesis.
In vivo
Although rodents express an SHBG homolog locally in the testis, the androgen-binding protein, hepatic expression of SHBG, and SHBG secretion into circulation are absent postnatally. This deficit in circulating SHBG explains low levels and susceptibility to the fluctuation of T in rodents [46]. As such, to study the regulation of SHBG expression in vivo, a mouse model expressing a human SHBG transgene has been developed [47]. These transgenic animal models expressing human SHBG had higher concentrations of T compared to wild-type mice [47]. In more recent work from our research group, SHBG concentration in SHBG transgenic mice has been shown to increase with age and is remarkedly higher in males. In addition, total serum T and DHT are increased approximately 200-fold as opposed to wildtype and multiple T precursors (e.g., pregnenolone, androstenedione) were also found to be increased [45]. Curiously, although androgen levels were raised in SHBG transgenic mice, free T concentrations, measured directly by liquid chromatography with tandem mass spectrometry (LC-MS/MS) following equilibrium dialysis (ED), were not different compared to controls. However, despite similar free T levels in SHBG transgenic mice, luteinizing hormone (LH) levels were significantly higher than in controls. Moreover, following orchiectomy and implantation of a continuous-release T implant, free T was lower in transgenic compared to wild-type mice [45]. With these findings, we provided evidence that the rise in LH was explained by an SHBG-mediated decrease in free T, thereby triggering hypothalamic-pituitary feedback. The activated axis in its turn regulates and again raises free T concentrations by increasing testicular T production. Ultimately, free T and not total T is apparently the biologically active signal which directly feedbacks the hypothalamic-pituitary axis.
Phenotyping of the SHBG transgenic mice identified a partially compensated hypogonadism reflected in an increased LH together with a minor, but significant, weight reduction of the seminal vesicles and pelvic bulbus cavernosus and levator ani muscles. Despite an extremely high amount of total T in these animals, weight reduction of the most androgen-sensitive organs provides in vivo evidence of androgen deficiency attributed to the inhibitory effects of high levels of SHBG [45]. More profound, administration of anabolic doses of T or DHT led to seminal vesicle hypertrophy in wildtype mice that is, however, prevented by SHBG in transgenic mice. Next to the androgen-suppressing effect of SHBG, injection of castrated mice with T, DHT, or mibolerone (a synthetic androgen with negligible binding affinity for SHBG) revealed that SHBG prolonged the circulating half-life of T and DHT, but not of mibolerone [45]. This confirms that SHBG has the capacity to bind and lengthen the half-life of its ligands.
In summary, our group contributed a considerable amount of data from in vitro and in vivo experiments that lend direct and convincing support to the core statements that make up the free hormone hypothesis. First of all, the ability of SHBG to suppress androgen bioactivity was confirmed in both in vitro and in vivo studies. Second, this suppression is advocated by high-affinity binding between SHBG and T, thereby rendering the SHBG-T complex biologically inert. Free T on the other hand appears to be able to cross the cell membrane and carry out its androgenic biological effect. Finally, regulation of this non-SHBG-bound fraction is under the tight control of a negative feedback loop through the hypothalamic-pituitary axis. These arguments underline the physiological importance of SHBG and brand it as the gatekeeper of intracellular concentrations of T and its biological action in target tissues.
Absence of binding proteins in human subjects favors biological activity through free hormones
Apart from in vitro and in vivo data, compelling human data in support of the free hormone hypothesis is also available. As discussed above, BPs are intrinsically important in establishing and maintaining the equilibrium between the protein and the non-protein-bound fraction. In fact, BPs themselves bear evidence in favor of the free hormone hypothesis. A family with a missense mutation within the SHBG gene was identified, which resulted in unmeasurably low SHBG concentrations in plasma. SHBG-deficient serum was obtained from a male subject in this family who suffered from low libido and erectile dysfunction, symptoms related to decreased T. This person, however, had normal male gonadal and pubertal development as well as reproductive function. Despite his extremely low total T, the biochemical analysis uncovered normal free T [48]. The absence of elevated LH in this individual indicated a normal pituitary perception of T availability. The subject's symptoms (e.g., low libido and erectile dysfunction), possibly due to late-onset hypogonadism, could in this case not be completely explained by T deficiency alone, since free T was normal. An analogous finding recently emerged for DBP and vitamin D. A patient was found to have a novel pathogenic variant of the GC-gene, encoding for DBP, resulting in undetectable levels of DBP but without any clinical signs of vitamin D deficiency. Biochemical evaluation of vitamin D metabolites indicated lowered values of total 25-hydroxyvitamin D (25(OH)D) and 1,25-dihydroxyvitamin D (1,25(OH)2D), but normal range values of the corresponding free fractions of these metabolites [49]. Both clinical cases provide support for the free hormone hypothesis and deliver additional arguments for the relevance of free metabolites as preferred indicators for clinical manifestations of hormone deficiencies.
The impact of variability in SHBG on free hormones
*Life cycle evolution of SHBG levels
As mentioned previously, free hormone concentration not only depends on the binding affinity of a given hormone for its carrier proteins but also on BP concentrations. SHBG levels change during different stages of human life [50]. SHBG can be measured in fetal blood and amniotic fluid from 13 to 20 weeks of gestation and does not significantly differ at term (36–37 weeks) [51]. Human SHBG levels do not differ between male and female fetuses [52, 53]. Maternal SHBG levels on the other hand are subject to a significant five- to tenfold increase from early to mid and late pregnancy [54]. This gain in SHBG might serve as a mechanism to protect the mother against the pregnancy-induced increase of androgens [13]. This assumption seems indeed confirmed by a rare observation of a woman with extremely low SHBG, caused by a combination of two rare single nucleotide polymorphisms (SNPs), who exhibited symptoms of hyperandrogenism during pregnancy, whereas the twin daughters she gave birth to did not show any signs of virilization [55].
In neonates, SHBG increases shortly after birth, attributed to a parallel postnatal increase in thyroid hormones [56, 57]. This increase persists and SHBG remains high throughout childhood. During puberty, the concentration of SHBG falls in both sexes, with a stronger twofold decrease in men, resulting in adult men having only half of the SHBG concentration as compared to women of the same age [58, 59]. The reason for this remarkable decline during puberty remains poorly understood. In the last decade, however, several factors have been associated with this decline in SHBG. In a large cross-sectional study of 903 healthy subjects aged 6 to 20 years, body mass index (BMI), T, estradiol, and DHEAS were found to be negatively correlated with the evolution of SHBG levels from prepuberty into adolescence in men. In women, however, this negative association with SHBG was only observed for BMI and DHEAS [60].
In contrast to declining SHBG levels throughout male puberty, androgen concentrations increase. However, in adult and elderly men, the opposite occurs: SHBG concentrations increase whereas androgen levels decline. Indeed, the European Male Aging Study (EMAS) showed different cross-sectional age trends concerning SHBG and androgen status in community-dwelling men aged 40–79 years [61]. While SHBG is significantly higher in older age groups, total T and free T both display a decreasing trend with older age. This negative trend is, however, markedly stronger for free T, with a decrease of 1.3% per annum as compared to a decrease of only 0.4% per year for total T. These observations of decreased androgen levels and increased SHBG have been confirmed in the longitudinal Siblos Extension Study (SIBEX) [62]. In conclusion, age is an important contributor to variance in total T, free T as well as SHBG [61, 62].
*Genetic variability in SHBG influences hormonal status
Production of SHBG is a complex process that is influenced by hormonal, nutritional, metabolic, and pharmacologic factors [63, 64]. Additionally, interindividual variation in SHBG levels has been shown to be highly heritable (between 30 and 80%). SNPs are important coding and/or regulatory sequence modifications in the SHBG gene that alter transcription, translation, and thus the production of SHBG in general. These SNPs can explain the relationship between genetic background and interindividual variation of SHBG and by extent, sex steroid status. Various polymorphisms in the SHBG locus and the X chromosome have been linked to substantial variations in SHBG and/or T concentrations (Table 2). Analysis of genome-wide association data of more than 8000 men identified that two SNPs within the SHBG locus are independently associated with serum T concentrations. Rs12150660, located 11.5 kb upstream of the major transcription start site of SHBG, represented the SNP with the strongest genotype-dependent difference in T. Mean serum T concentrations are found to be highest in men with the TT genotype, lowest for the GG genotype and situated in between for the GT genotype of this SNP. For the second SNP, rs6258, a rare missense polymorphism located in exon 4 of SHBG, lower concentrations of T were observed for the CT vs CC genotype. Additionally, as rs12150660 and rs6258 are located within the SHBG locus, a relevant association with SHBG concentration was demonstrated for both SNPs [65–67]. Differences in serum T concentrations for both SNPs, however, were still significant after adjusting for these altered SHBG concentrations [65]. Furthermore, the CT genotype for the rs6258 polymorphism results in reduced binding affinity of SHBG for testosterone. In fact, because of the lower binding affinity of SHBG, the percentage of free T was 22% higher in individuals with the CT vs the CC genotype [65]. For a third SNP in the SHBG locus, rs727428, the T allele accounted for a decrease in both SHBG and T levels, without affecting calculated free T [66, 68].
Important genetic polymorphisms have also been identified in the promotor region of SHBG, adjusting its expression levels. First, total T, and not free T, was elevated in subjects with six repeats, as compared to seven or more, of the (TAAAA)n repeat polymorphism. This effect occurred independently from SHBG levels [69]. Other studies confirmed this observation, although their data also suggest an increase of free T in subjects with nine or more repeats [70, 71]. Furthermore, the number of (TAAAA)n repeats was also inversely related to SHBG levels [69, 70]. Second, for the rare homozygous AA genotype of rs1799941, an increase in both serum SHBG and T was observed as opposed to the reference genotype [66, 69, 72, 73]. Although more recently the same AA genotype of rs1799941 was linked to lower calculated free T [74], this was not preceded by findings in earlier studies [66, 69, 73]. Finally, for carriers of two other SNPs, rs6257 and rs6259, a decrease and, respectively, an increase of SHBG was observed [75].
Similar to the polymorphisms specified above, all located on chromosome 17 within the SHBG gene or its promotor, associations to serum androgen levels have been reported for loci on other chromosomes. Rs5934505 is an SNP located at Xp22, positioned 79 kb downstream and 145 kb upstream of, respectively, FAM9B and FAM9A (family with sequence similarity 9, member B and A), two genes that are expressed exclusively in the testis [76]. FAM9A and FAM9B, are believed to be involved in, among other, the formation of the synaptonemal complex and DNA replication/recombination during cell division. Nonetheless, the physiological importance of these genes remains largely unclear [76]. In men presenting with a T versus C allele of rs5934505, a decrease in total and calculated free T, but not in SHBG concentrations was observed [65, 77]. Another SNP, rs10822186 at 10q21, has also been associated with changes in T levels in men. This locus was connected to JMJD1C, a gene that might be involved in testosterone synthesis. A genetic variation in this gene may cause a change in circulating androgen levels [77].
Analogous to SHBG, comparable experiments have been carried out for DBP. As the major carrier for vitamin D in circulation, it serves a primordial role in determining total and free concentrations of vitamin D [3]. Similar to SHBG, over 120 genetic variants of DBP have been discovered of which three major polymorphic alleles are the most relevant ones (GC1f, GC1s, and GC2), yielding six allelic combinations and three corresponding phenotypes [78]. A variation in geographical distribution is seen in these phenotypes, as for instance in Africans, GC1f is the most common allele while in Europeans GC1s is most frequently found [79]. This genetic variation has been deemed the main argument for the observation of lower levels of both DBP and 25(OH)D in subjects of African versus European origin, while levels of bioavailable 25(OH)D were similar in both ethnic groups [80]. However, as no racial difference in DBP was observed when using a polyclonal assay or applying LC-MS/MS, this conclusion was most likely the result of a monoclonal assay bias, confirming poor vitamin D status in subjects of African origin [81, 82].
Regulation of SHBG levels
Biosynthesis of SHBG is mainly situated in hepatocytes and transcriptional control of the SHBG gene is, as discussed in the previous section, influenced by various factors [83, 84]. A mouse model with a gene addition of the 4.3 kb human SHBG uncovered that upstream of exon 1, a sequence of approximately 0.9 kb suffices for high gene expression levels [47]. Further characterization of this upstream region identified binding sites for chicken ovalbumin upstream promoter transcription factor (COUP-TF) and hepatocyte nuclear factor-4 alpha (HNF-4α) [85]. Competing for a mutual binding site, HNF-4α and COUP-TF create a functional antagonism, respectively, actively recruiting or blocking the transcriptional machinery, which is essential for the expression of SHBG in the liver. Their interaction serves as a molecular ‘on-off switch’ mechanism that explains the acute response of SHBG expression to changes in metabolic states [85].
Levels of SHBG in circulation are governed and affected by several determinants, summarized in Table 3. A consistent finding is a negative association between SHBG and BMI [86, 87]. Other indices used in the assessment of central obesity, such as waist circumference and waist-hip ratio, also exhibit this negative association with SHBG [88]. Indeed, male and female subjects suffering from obesity show lower levels of SHBG compared to their non-obese peers [87, 89]. In fact, a negative association between obesity and SHBG is even present in overweight and obese children [90]. Moreover, following bariatric surgery, SHBG levels rapidly increase [91, 92]. Furthermore, this relationship between plasma SHBG levels and obesity is stronger than the positive association of SHBG with age described earlier. SHBG concentrations are highest in non-obese older men and lowest in obese younger men [87]. In contrast, in female patients with anorexia nervosa, SHBG concentrations were lower compared to normal weight subjects and increased to the normal range after adequate weight gain, thus being a reliable index of nutritional status [93]. Apart from BMI, other conditions influence SHBG levels. For instance, in both sexes, HIV-positive individuals have increased SHBG concentrations compared to their seronegative peers [94–96]. Thyrotoxicosis is also featured by an increase in SHBG as well as increased total T concentrations [97]. Hypothyroidism on the other hand is accompanied by a decrease in both SHBG and total T [98], which is reversible with adequate treatment [99].
Fluctuation of SHBG can also arise from the administration of exogenous substances that display estrogenic and/or androgenic properties. Among others, estrogen-containing products, like combined oral contraceptives (COC) but not their progesterone-only counterparts, are associated with an increase in SHBG concentration and other major changes in several plasma protein profiles [100]. Tamoxifen, mainly used in the treatment of breast cancer predominantly displays an estrogen-like effect on hepatic tissue, stimulating SHBG production [101, 102]. Similarly, the use of mitotane in the treatment of adrenal carcinoma is associated with an increase in plasma SHBG levels [103]. Contrary to drugs with estrogenic effects, danazol, mainly used in the context of endometriosis, is a compound of androgenic nature. Binding to SHBG with high affinity, it is capable of altering the binding affinity of SHBG for T thereby displacing it from its carrier, subsequently increasing free T, and eventually leading to decreased concentrations in SHBG [104–106]. Affecting sex steroid balance in a different way, drugs without innate estrogenic and/or androgenic properties also exert their effect on SHBG levels. Administration of some antiepileptic drugs (e.g., phenobarbital, phenytoin, and carbamazepine) can induce hepatic microsomal enzymes. This results in an increase in SHBG concentration that in turn influences free circulating androgen levels, lowering T bioavailability [107–109]. Administration of statins is associated with a decrease in SHBG [110, 111]. In another example, treatment with synthetic glucocorticoids, for instance, dexamethasone, has also led to decreased SHBG levels in women with PCOS and in children with acute lymphoblastic leukemia [112, 113]. Clearly, SHBG levels in circulation respond to different endocrine and metabolic signals. This in turn affects circulating T levels and hence designates SHBG as a potential biomarker for the disease.
SHBG: a potential biomarker of disease?
In the last decade, SHBG has been the center of attention in research on type 2 diabetes mellitus (T2D), obesity, PCOS, male hypogonadism, liver disease, bone health, and even cancer. All these disorders have been associated with altered SHBG levels [114]. As a result, the clinical usefulness of SHBG and its potential as a biomarker of disease has been questioned. To assess this potential, the classical definition of a biomarker is of great importance: “A defining characteristic that is measured as an indicator of normal biological processes, pathogenic processes or responses to an exposure or intervention [115].” Additionally, to be able to classify SHBG as an ideal marker, among others it should be specific to a particular disease and able to differentiate between different physiological states [116]. For the following reasons, SHBG fails to meet this qualification. First, almost all SHBG associations result from cross-sectional study designs. As no prospective studies have been conducted, statements on causation are lacking. Second, SHBG shows a strong correlation to T, making it difficult to observe an independent SHBG effect. Finally, and vice versa, designing a study to reveal an independent effect of SHBG proves to be challenging, as SHBG and T are tightly bound. In summary, while SHBG may not classify as an ideal diagnostic biomarker, it shows associations with different disorders. As such, and for further research purposes, we deem it useful to provide a concise overview of these associations.
*Glycemic control and progression to metabolic syndrome
SHBG has been implicated as a contributing factor to T2D and insulin resistance (IR), entities closely related to obesity
*All previous data show a convincing association between obesity/BMI, T2D, METS, and SHBG. Focusing on metabolic disturbances, the inclusion of SHBG in diagnostic evaluation protocols could be an asset.
*SHBG in nonalcoholic fatty liver disease
Nonalcoholic fatty liver disease (NAFLD) is a common cause of chronic liver disease, possibly progressing to cirrhosis or hepatocellular carcinoma. NAFLD is mainly characterized by the accumulation of triglycerides and fatty acids in the liver, in the absence of a secondary cause of fatty liver such as excessive alcohol use or viral hepatitis.
*While the link between SHBG and NAFLD progression is not yet fully elucidated, SHBG clearly shows associations with NAFLD.
*SHBG and bone health
A link between SHBG levels and osteoporosis has also been reported. [145].
*All of these studies, both in men and in women, suggest an association between SHBG, bone health, and fracture risk.
Diagnosing hypogonadism in men: total versus free testosterone
Male hypogonadism is a clinical condition that can be subdivided into two types. Organic hypogonadism is characterized by a structural, congenital, or destructive disturbance of the hypothalamic-pituitary-gonadal (HPG) axis, leading to the inability of the gonads to produce a sufficient amount of androgens resulting in clinical signs of androgen deficiency. Functional hypogonadism on the other hand refers to identical clinical signs and coexisting androgen deficiency without an intrinsic pathology of the HPG axis [165]. These androgens are, during the male life span, needed to promote masculine development/virilization and growth, and are of vital importance in reproductive functioning [166]. Manifesting differently between male individuals, symptoms of androgen deficiency vary according to the age of onset, the severity of T deficiency, androgen sensitivity, and previous and/or current use of T replacement therapy [166]. These symptoms cover a broad clinical spectrum (Table 4). It is, however, clear that a number of these complaints overlap with other chronic diseases such as obesity and diabetes mellitus. In fact, multiple studies confirmed the association between low or inappropriate T levels in men and T2D and obesity [167–170]. Furthermore, because of the high prevalence of cooccurrence of T2D and obesity, low T was unsurprisingly also associated with metabolic syndrome [171, 172]
While these clinical symptoms are the main motivation for patients to visit a physician, the diagnostic emphasis of androgen deficiency has heavily shifted onto biochemical measurement of circulating T. Confirmation of deficient gonadal production of T is, therefore, reflected in the measurement of low total T in serum [173]. Despite being the first-line test in the diagnosis of hypogonadism in all practice guidelines, there is no agreement between these guidelines concerning differentiating low from normal circulating T (Table 5). Although there are no issues concerning test sensitivity and picking up low-range concentrations of total T, a precise definition of the lower limit of ‘normal’ total T remains ambiguous. Multiple studies attempted to establish a reference range to achieve generally accepted values for total T in young men, ending in diverse intervals for all different cohorts [174–177]. A collaboration of European and American researchers and clinicians argued that these differences could be attributed to geographic region, interlaboratory assay validation as well as biological and environmental factors. In their effort to harmonize reference ranges, they established 264 to 916 ng/dL as a normal interval for total T in European and American non-obese men aged from 19 to 39 years. Using a reference method from the Centers for Disease Control and Prevention (CDC) they concluded that previous cohort inter-variability was mainly due to a substantial amount of inter-assay variation [178]. The use of this reference method should allow for interlaboratory harmonization of total T reference ranges in men. Caution, however, is still advocated when classifying men according to total T values. Currently, obesity is one of the leading major health problems, with over 650 million people affected worldwide [179]. Imposed by their obese status, these men are prone to lower total T as well as lower SHBG concentrations, implying a substantial risk for misclassification when using ‘normal’ reference ranges [180, 181].
While we agree that the measurement of total T is and should remain the first step in diagnosing male hypogonadism, the results of an observational study conducted by our research group confirmed the potential added value of free T in this context [182]. In our study, despite men having normal total T levels, a low calculated free T serum level was associated with androgen deficiency-related symptoms (sexual, physical, and psychological functioning, biochemical measurements, body composition, and bone measurements). Moreover, cases of low total T in combination with normal calculated free T were not associated with hypogonadal signs. With regard to our results, we recommend that free T should be included on top of total T in the evaluation of hypogonadism in men, especially in obese subjects [182]. Moreover, in HIV-positive men, underdiagnosis of male hypogonadism would have occurred in one-third to half of the study subjects if only total T was measured as opposed to combining it with free T [183, 184]. These data underline the importance of free T values and adding this parameter to biochemical testing could, therefore, increase the diagnostic sensitivity of male hypogonadism. Using this knowledge, the Endocrine Society (ES) decided to amend its guidelines on the diagnosis of hypogonadism in men, including free T as a novel parameter [7]. In the diagnostic approach proposed by the ES, total T should be measured in a fasting subject on two separate mornings. Additionally, determining free T is either advised when men are subject to conditions that alter SHBG levels or when the initial measurement of total T was below or close to the lower limit of the normal range. Furthermore, recommendations on determining free T are either direct measurement by ED or through a calculation using total T, SHBG, and albumin values [7].
Free sex steroids in women with polycystic ovarian syndrome
PCOS is one of the most common endocrine disorders worldwide, with, depending on the criteria used, a prevalence rate of 4% up to 18% [185]. PCOS leads to infertility, menstrual problems, and clinical manifestations of hyperandrogenism in women of childbearing age [186]. Women with PCOS are often obese, develop IR, and are at risk of developing T2D [187, 188]. The pathological mechanism by which PCOS arises is very complex, and multifactorial, and still remains largely unclear, but appears to be a disbalance in hormones, androgens, and insulin in particular [189]. Furthermore, obesity may exacerbate features of PCOS by worsening IR and stimulating hyperandrogenism [190, 191].
In the diagnostic workup of PCOS, androgen excess is usually assessed by measurement of total T [187]. In routine clinical practice, total T is most frequently measured by immunoassay methods. However, some caution is warranted as commercially available immunoassays may not be suited to accurately and precisely determine low T concentrations in women [192]. As a consequence, total T by direct immunoassay misclassifies PCOS in 1 out of 3 subjects [193]. Hence, the acquisition of total T results in women should be done by chromatography-mass spectrometry, which has become the gold standard for the measurement of sex steroids [39, 187]. Notwithstanding more accurate total T results can be achieved with LC-MS/MS, total T may be an insensitive marker for hyperandrogenism in women [193]. In contrast to total T, free T seems to be a better marker to determine hyperandrogenemia in women with PCOS [187], provided that an accurate and high-quality methodology is used for measured or calculated free T [193, 194].
Together with free T, SHBG has recently been recognized as a useful diagnostic marker and therapeutic target for managing women with PCOS [195]. A meta-analysis showed that subjects with PCOS had remarkably lower levels of SHBG and that this effect was even more pronounced in subjects with concomitant obesity [196]. In the evaluation of androgen excess in women suspected of PCOS, it is thus important to evaluate both free T and SHBG, as they reflect both metabolic and ovarian disturbances associated with this disease [197].
Methodology on calculated and measured free testosterone
Provided the evidence that free T gains clinical importance in the diagnostic landscape of male hypogonadism, several methods for the assessment of this free fraction have been developed and are currently available. Moreover, not only can free T be of value in the clinical context of hypogonadism but, as discussed in another section of this review, due to alterations in SHBG and total T, free T might be of interest in several other pathologies. In the clinical setting, assessment of free T can be carried out either by direct measurement or through calculation.
Measurement of free testosterone
Measurement of serum-free T through ED is, as recommended by ‘The Princeton Consensus Statement’ and many other publications, the well-established reference method [198]. This technique allows for dialyzing a patient’s serum or plasma across a semipermeable membrane, retaining protein-bound T in the process. Whereas SHBG and albumin-bound T are withheld from the dialysate, unbound (free) T can freely pass. After reaching equilibrium, the dialysate can subsequently either be analyzed by a direct or indirect method, thereby describing the technique as direct or indirect ED. In direct ED, measurement of the free hormone concentration in the dialysate is directly performed by LC-MS/MS. Indirect ED on the other hand uses a labeled (usually tritium) tracer steroid, and the measured total steroid concentration, to proportionally determine the free steroid concentration. Indirect ED, as compared to direct ED, is subject to several limitations, such as tracer instability, tracer purity, and the need for a dilution step, that all introduce possible sources of added total error to the free steroid measurement [199, 200]. Hence, direct ED using LC-MS/MS is the preferred method for the measurement of free serum hormones.
Different other methodologies for assaying free fraction steroids exist, but these are beyond the scope of this review. Briefly, LC-MS/MS coupled ultrafiltration (UF) constitutes a comparable alternative to ED. UF has also previously been described in the validation of a reference measurement procedure for free T [200]. Little data exist on the validation of UF versus ED, yet UF is a relatively convenient technique and when properly validated can display equal performance to ED [199, 201, 202]. Similar to ED, UF deals with its own technical issues. The most common pitfalls of UF are due to non-specific binding, steroid adsorption to the filtration membrane, and protein leakage through the filtration membrane [200, 203]. Additionally, an analog-based immunoassay has been developed. These methods claim to measure free hormones directly in serum. Nevertheless, inadequate accuracy, bias, and dependency on T rather than free T are major drawbacks in these analog-based assays [204–206]. Comparative studies of this analog-based immunoassay and ED confirmed that this technique does not detect nor quantify free T and that it should, therefore, not be used as an assay for free T [205, 207, 208]. This led the ES to recommend against the application of this assay in clinical settings [39]. While the use of this analog-based technique is clearly decreasing, surprisingly, a survey conducted in Northern America acknowledged that in 2011 approximately 33% of queried clinical laboratories still used this analog-based test as the primary test to measure free T [209].
As mentioned before, it is not only possible to assess the free fraction, but also the bioavailable fraction of sex steroids. The bioavailable fraction of T consists of both the free T fraction and the albumin-bound T fraction. In this definition of bioavailable T, the albumin-bound fraction is designated part of the usable T pool because of the low Ka between T and albumin, making the binding between ligand and carrier prone to dissociation [21, 22]. The gold standard for measurement of bioavailable T is a direct precipitation method with either ammonium sulphate or concanavalin A. Both substances form a complex with SHBG, resulting in precipitation of the carrier and by extension, the SHBGbound T. Addition of a tracer element, 3 H-testosterone, to the sample or direct measurement by immunoassay or LC-MS/ MS, allows for assessment of both the free and albumin-bound fraction in the supernatant [208, 210]. However, this method is rarely used in diagnostic laboratories as it is not automated, is cumbersome to execute, and may involve radioactive tracer elements. Furthermore, because it is not standardized, large variability in bioavailable T results may exist between laboratories [208]. Additionally, the measurement or calculation of bioavailable T is not mentioned in any clinical guidelines [6, 7]. As such, bioavailable T is only used in a limited way and evidence of clinical associations is scarce. For now, we would not recommend using bioavailable T as an alternative to free T.
Calculation‑based free testosterone
Because of the limited availability of ED in clinical settings and the unsuitability of direct free testosterone assays, values for free T are frequently obtained through calculation. Multiple calculation algorithms, using other serum-measured biochemical variables, e.g., SHBG, albumin, and total T concentrations, have been computed. These formulas depend on the precision and accuracy of the methodologies of their included variables. Immunoassays for total T; SHBG and albumin are validated diagnostic techniques. Despite their high availability and improvements over the last 30 years, performance issues with regard to precision and accuracy still exist. On the other hand, LC-MS/MS provides a more accurate measuring method for total T [39, 211]. A wide spectrum of algorithms for free T exists, which can broadly be divided into three categories (Table 6). First, several equations were derived from the general law of mass action. Association constants for albumin and SHBG used in these formulas were obtained from in vitro experiments by linearizing data through Scatchard analysis [16, 208, 212]. A second category contests the principle of Scatchard analysis, refuting the assumption that SHBG expresses linear binding kinetics [213]. This model takes into account allosteric interaction between binding sites for T on the SHBG homodimer. Empirical models exploiting bootstrap regression methods on cohort datasets comprise the third and last category [214, 215]. All these models have respective strengths and intrinsic limitations, as described in an extensive review [5].
An alternative method for free fraction T calculation is the use of the free androgen index (FAI). The FAI is calculated by the ratio of total T to SHBG, delivering an alternative way of estimating free androgens. Because of its accessibility, the FAI is widely utilized in clinical laboratories and clinical studies spanning different disciplines in medicine [216, 217].
Additionally, in many research designs on hyperandrogenism in women, FAI is a key parameter of interest [218, 219]. Caution, however, should be exercised, as the FAI is highly impacted by SHBG concentrations. In human subjects, male or female, in whom SHBG concentrations are altered, FAI could increase or decrease dramatically. This results in an artificial over- or underestimation of free fraction androgens [220]. As disserted previously in this review, SHBG concentrations are subject to change in pathologies such as obesity, diabetes, and metabolic syndrome. It is, therefore, not advised to use FAI as a clinical decision-maker.
Agreement between measured and calculated free testosterone
Regrettably, all the methods described above leave researchers and clinicians with the critical question of which method provides the best fit to delimit free T values. To address the matter, a Belgian research consortium including some of the authors of this review, assayed over 300 serum samples of human males and females for free T [221]. Measurement of free T on all samples was performed by gold standard LC-MS/MS coupled ED. In addition, free T for each sample was calculated using the Vermeulen, Zakharov, and Ly/ Handelsman models. Median bias, expressed by the ratio of calculated to measured free T, was 1.19, 1.0, and 2.05 for the Vermeulen, Ly/Handelsman, and Zakharov models, respectively. Our data also demonstrated that both Ly/Handelsman and Zakharov methods do not operate independently of SHBG, total T, and to a lesser extent albumin values [221]. This is particularly important in distinct conditions where SHBG and total T are in the lower range, increasing the probability of underestimating free T by calculation. Underestimation may pose a problem, especially in clinical settings of hypogonadal men (low total T), obesity (low SHBG), and women with PCOS (low SHBG). Paradoxically, assessment of these patient groups is also commonly the most relevant reason for requesting free T. In contrast we concluded that, although overestimating free T by 20–30%, the formula according to Vermeulen was independent of SHBG, total T, and albumin [221]. Taken into consideration the moderate bias level, but most importantly less influenced by the latter three variables, the Vermeulen formula, for the time being, still appears to be the most robust approximation and deserves our recommendation as a free T calculator for clinical use.
Free testosterone in clinical practice: limitations and approaches
Direct measurement of free T using LC-MS/MS coupled ED is currently, as previously discussed, the gold standard. Essentially obtaining accurate results, execution of this assay is, however, very labor intensive and technically challenging, resulting in limited availability of the method in everyday clinical routine [222]. In fact, currently non-automated, the process is subject to operator-dependent errors. Apart from these technical difficulties, assay performance is also affected by various conditions and error sources, governing high assay variability. Adhesion of components to the membrane or the dialysis chamber, dilution, assay temperature, and buffer characteristics such as volume, pH, and composition all impose differential effects on binding parameters and equilibrium conditions leading to distinct quantifications [39, 200, 208, 223–226]. In addition to these technical issues, the deployment of an ED technique adds extra costs to clinical laboratory practice [227]. As such, LC-MS/MS coupled ED is mostly only available in academic laboratories. Additionally, the measurement of (free) T is of less commercial interest, unlike (free) thyroid hormones. Whereas thyroid diagnostics make up a large part of daily laboratory tests requested by almost all clinicians, T is mostly only requested by endocrinologists and urologists. Hence, in the past decades, more resources have been invested in clinical thyroid diagnostics, enabling the commercialization of kits for the clinical laboratory allowing direct measurement of free thyroid hormones. In contrast, fewer funds were, and still are, directed at the development of assays for free T.
There is, however, a fairly good agreement between measured and free T calculated by the Vermeulen formula, if validated against ED [221, 228]. In fact, in clinical settings where direct measurement of free T is not available, the Vermeulen formula can serve as an acceptable clinical alternative. In addition, calculation as opposed to direct measurement also presents as a more convenient and cheaper quantitative metric. Summarized in Table 7, users of free T calculators should on the other hand also consider the different imperfections of these clinical utilities. Most importantly, in these models, free T is calculated through values for SHBG, albumin, and total T. Consequently, calculation quality depends on the performance characteristics of the assays used to determine these three variables. Second, concerning the interaction between SHBG and T, variability and assumptions in terms of binding affinity, stoichiometry, and allosteric interaction produce different quantities of free T in different models [5, 208]. Third, all of these models are in fact simplified versions of the true binding milieu and may potentially not include all variables affecting the equilibrium between total and free T (e.g., the role of CBG). Finally, for both measured and calculated free T, reference ranges may not be universally valid. Depending on the method to assess free T as well as the characteristics of the cohort, different intervals may be generated [177, 229]. In extension, there is no consensus on a universal cut-off between low and normal values for free testosterone. Further research efforts on harmonizing these reference ranges are, therefore, needed.
*In summary, we would suggest a pragmatic and practical approach to assessing androgen status in a clinical setting. Measurement of total T and SHBG is standard of care, which in any case also allows for the additional calculation of free T. In an altered SHBG-binding milieu, or when total T is borderline normal or below the lower limit of normal, accurate determination of free T can subsequently be carried out using LC-MS/MS coupled ED
Linking clinical endpoints to free testosterone
*Androgen‑related clinical endpoints
Illustrated in the previous sections of this review, free T has its role in the diagnosis of male hypogonadism. In the assessment of the quality of life in hypogonadal patients, physicians mostly rely on androgen-dependent clinical endpoints. While these endpoints have been profoundly studied in terms of total T concentrations, evidence on the link between free T and these endpoints is more scarce. Interestingly, while demonstrated that, in the case of the Vermeulen formula, calculation of free T leads to a constitutive positive bias of 20–30% compared to direct measurement using ED, almost all current research uses one of the previously mentioned calculation models to study associations with clinical endpoints.
*Clinical outcomes in other diseases
In related research, calculated and/or measured free T, as opposed to total T, were found to correlate stronger with clinical outcomes of NAFLD, PCOS, and hypertension [143, 237, 238].
*Although free T has been clearly associated with several clinical endpoints of interest, the fact that these conclusions are based upon calculated, and not directly measured, free T is a less potent element of the free hormone hypothesis statement. As discussed before in this review, free T calculations have several limitations. However, repeating these clinical studies using direct ED measurement would impose technical and logistical challenges, as well as require a great deal of effort from all scientific collaborators. While, however, not available at this moment, endeavors are underway to optimize the implementation of free T in clinical practice.
The need for free testosterone in the clinical setting: why and when?
Men with established organic hypogonadism are usually treated with T replacement therapy. Available in various formulations, androgen replacement serves the goal of establishing and/or sustaining secondary sexual characteristics in these men. Detailed specifics on administration, intended and adverse effects of T replacement therapy are beyond the scope of this review and have been discussed elsewhere [166]. Prescription of T supplements has increased phenomenally during the past 2 decades, especially in the larger group of men suffering from functional hypogonadism [247]. Nevertheless, T supplementation is not without risk and could potentially be harmful concerning cardiovascular risk in the elderly [248]. Currently, the path to diagnosing hypogonadism is still directed towards biochemical dosing of total T. However, conditions that affect SHBG levels unequivocally affect total T concentrations. Free T on the other hand is not necessarily impacted by this change in SHBG. The question rises of how free T can be integrated into a clinical workflow concerning male hypogonadism. More systematic use of free T on top of total T could reduce overtreatment and T prescriptions.
*With these combined results of the cross-sectional and longitudinal analysis, we clearly highlight that the sole use of total T could lead to either under or overdiagnosis of hypogonadism. To avoid these pitfalls of misdiagnosis, in particular when total T is borderline low and/or SHBG concentration is altered, the addition of free T to the diagnostic landscape of male hypogonadism is of incontrovertible value.
Conclusion
This review delivers strong arguments from in vitro, in vivo, and human experiments in support of the free hormone hypothesis. While we also acknowledge particular shortcomings in the hypothesis, we believe free fraction hormones as described by the free hormone hypothesis provide clinical utility in patient healthcare. Assessing free T on top of total T as a standard biochemical parameter in the diagnostic workflow of male hypogonadism is clinically relevant when total T is borderline low and/or SHBG concentration is altered. Furthermore, the measurement of free T in PCOS and other endocrinological conditions can be of diagnostic value. As LC-MS/MS coupled ED is not routinely available, for now, calculated free T by means of the Vermeulen formula is preferential to assess andrological status in the clinical setting. However, several barriers remain in the implementation of free testosterone into routine clinical practice (Table 8). More in-depth research is needed on the interaction between steroid hormones and their BPs. Further exploration of the steroid binding milieu and development of corresponding models (e.g., reappraisal of free hormone calculators) can establish the clinical relevance of these free fraction hormones. In addition, efforts should be made to develop new or improve existing free hormone methodologies. Improving availability and accessibility to direct measurement methods are important milestones and will deliver added value to the diagnostic landscape of hormonal exposure in various endocrinological conditions.
2018
academic.oup.com
N. Narinx · K. David1, · J. Walravens · P. Vermeersch · F. Claessens · T. Fiers · B. Lapauw · L. Antonio, · D. Vanderschueren
Keep in mind this is Fier's camp (cFTV).
Nothing new that those in the know were already well aware of!
You heard it here first the one and only Nelson's ExcelMale!
Abstract
According to the free hormone hypothesis, the biological activity of a certain hormone is best reflected by free rather than total hormone concentrations. A crucial element in this theory is the presence of binding proteins, which function as gatekeepers for steroid action. For testosterone, tissue exposure is governed by a delicate equilibrium between free and total testosterone which is determined through interaction with the binding proteins sex hormone-binding globulin and albumin. Ageing, genetics and various pathological conditions influence this equilibrium, hereby possibly modulating hormonal exposure to the target tissues. Despite the ongoing controversy on the subject, strong evidence from recent in vitro, in vivo, and human experiments emphasizes the relevance of free testosterone. Currently, however, clinical possibilities for free hormone diagnostics are limited. Direct immunoassays are inaccurate, while gold standard liquid chromatography with tandem mass spectrometry (LC-MS/MS) coupled equilibrium dialysis is not available for clinical routine. Calculation models for free testosterone, despite intrinsic limitations, provide a suitable alternative, of which the Vermeulen calculator is currently the preferred method. Calculated free testosterone is indeed associated with bone health, frailty, and other clinical endpoints. Moreover, the added value of free testosterone in the clinical diagnosis of male hypogonadism is clearly evident. In suspected hypogonadal men in whom borderline low total testosterone and/or altered sex hormone-binding globulin levels are detected, the determination of free testosterone avoids under- and overdiagnosis, facilitating adequate prescription of hormonal replacement therapy. As such, free testosterone should be integrated as a standard biochemical parameter, on top of total testosterone, in the diagnostic workflow of male hypogonadism.
Introduction
Steroid hormones are biological signal transducers that are vital in coordinating diverse developmental and physiological processes. From fetal life into adulthood, steroid hormones regulate different important functional axes in the human body. Physiologically, steroid hormones are divided into three categories: sex steroids (androgens, estrogens, and progestogens), corticoids (mineralocorticoids and glucocorticoids), and vitamin D [1]. While all of these steroid hormones serve a different physiological purpose, their steroidogenesis all starts with the same molecule, cholesterol. Hence, structurally, each type of steroid hormone is based on the classic cyclopentanophenanthrene four-ring structure, or a derivative [2]. Because of their lipophilic properties, the transportation of steroid hormones to target organs and tissues via the bloodstream is mediated through binding proteins (BPs). In fact, the majority of steroid hormone molecules adhere to these BPs, leaving only a small fraction that circulates freely in human plasma [3]. The free hormone hypothesis delivers a rational approach to the biological activity of these different fractions, thereby redefining hormonal exposure in terms of free steroid hormones.
Notwithstanding emerging evidence on the subject, the free hormone hypothesis remains somewhat controversial [4]. Multiple elegant manuscripts reviewing free fraction hormones have been published in the last decade, each one conveying a slightly different message to its readers [3–5]. Accordingly, the debate around free testosterone (T), and its potential use in clinical settings, caused the formation of two movements. While the majority of societies on endocrinology have implemented free T into their guidelines on male hypogonadism, the Australian Endocrine Society does not recommend its usage for clinical decision-making [6, 7]. The purpose of this review is to update current views on the free hormone hypothesis using recent knowledge. In this review, we will highlight recent results from important in vitro, in vivo, and human studies from our group and others, thereby refocusing the use of free T and driving its implementation into the clinical context. As such, we will demonstrate the need for free T in both male and female patient populations, for example in the diagnosis of male hypogonadism and polycystic ovarian syndrome (PCOS). Furthermore, we will discuss the methodology for measured and calculated free T in great detail, including recent data showing that the Vermeulen formula deserves clinical recommendation as the free T calculator of choice. After highlighting the biological relevance and added diagnostic value of free T in the clinical setting, we will conclude with a research agenda giving an overview of open questions and obstacles yet to be cleared.
Steroid hormone‑binding proteins: gatekeepers of hormonal exposure
An essential factor to be considered in the free hormone hypothesis is the contribution of plasma BPs. In human steroid physiology, several BPs have been identified. Not only do these BPs provide the transport of steroid hormones from their production to their target site, but they also contribute considerably to the equilibrium between the free and protein-bound fractions in plasma. As such, BPs function as primary gatekeepers of steroid action [8].
Binding proteins define steroid hormone fractions
Production of steroid hormones occurs at different sites in the human body, delineating a specific process for each hormone. Following biosynthesis, transport to target organs and tissues is facilitated by a group of plasma BPs that are either specific or non-specific carriers. Specific steroid carriers include SHBG which preferentially binds T, dihydrotestosterone (DHT), estradiol, and other sex steroids, vitamin D-binding protein (DBP) for vitamin D and its metabolites, and cortisol-binding globulin (CBG) that preferentially binds cortisol, aldosterone, and progesterone. Additionally, thyroid-binding globulin (TBG) and transthyretin act as specific carriers for thyroid hormones. Next to specific transport, the most abundant human plasma protein, albumin, provides non-specific means of transportation for all previously mentioned components. As a result, three plasma hormone fractions can be defined: the non-protein-bound (free) fraction, the fraction bound to specific BPs (e.g., SHBG), and the albumin-bound fraction (Fig. 1). The free hormone concentration is determined by the level of total hormone, as well as the level of BP and albumin in circulation and the binding affinity of a hormone for its carrier(s), thereby characterizing the equilibrium state between the free and the bound fractions.
SHBG and albumin define a delicate equilibrium in testosterone fractions
The bulk of T transportation is operated by three carriers: SHBG, CBG, and albumin, respectively, representing 44, 4 and 50% of the total T fraction in human male plasma [9]. While the vast majority of circulating T is protein-bound, on average only 2% circulates freely [9]. Human SHBG is a glycoprotein that is mainly produced by the liver. It circulates as a 90–100 kDa homodimeric protein and is able to bind all androgens and estrogens, with the exception of dehydroepiandrosterone sulfate (DHEAS) and androstenedione. Recent studies on UK biobank data indicated that the median plasma SHBG concentration is 36.89 nmol/L in men. In women, median SHBG plasma concentration was valued at 60.34 and 53.56 nmol/L for pre- and postmenopausal age, respectively [10, 11]. This carrier protein is encoded by the SHBG gene, found on the short arm (p12-13) of chromosome 17, consisting of eight exons [12]. Besides expression in the liver, the gene is also transcribed in the brain, uterus, breast, ovary, placenta, prostate, and testis [13]. The binding of sex steroid metabolites is accomplished in a binding pocket of the homodimeric protein, formed through the association of two monomers, stabilized by the bivalent cations zinc and calcium, with each homodimeric protein holding up to two molecules. A serine residue at position 42 in the binding pocket is responsible for the formation of hydrogen bonds with functional groups of T and estradiol [5, 14, 15]. The dynamic relationship between T and SHBG is characterized by their binding affinity, a measure of the strength of interaction between metabolite and carrier. Among steroid hormones, T has the second highest association constant (Ka), valued at 1–2 × 109 M−1 s−1, whereas DHT has the highest Ka for SHBG (2.69 × 109 M−1 s−1) [16]. Therefore, binding between T and SHBG occurs rapidly and is very strong with little tendency for spontaneous dissociation. Binding affinities of SHBG, and all other previously mentioned BPs, have been subject to extensive experimental research, summarized in Table 1 [9, 16].
Albumin, designated as a non-specific transporter of T in the context of the free hormone hypothesis, is the most abundant protein in human plasma. It is a 66.5 kDa protein, circulating at a concentration of 35.0 to 50.0 g/L in both men and women, and is mainly responsible for maintaining colloid osmotic pressure [17]. It also plays a major role in the transportation and homeostasis of diverse ligands (electrolytes, fatty acids, hormones, vitamins, and drugs…) [18]. Synthesis of albumin only occurs in the liver, initiated by transcription of the ALB gene located on chromosome 4 (chr4q13.3) [19]. The binding affinity of T for albumin (Ka of±4× 104 M−1 s −1) is five orders of magnitude smaller and weaker than the binding affinity for SHBG. However, genetic variation in albumin could contribute to altered plasma albumin concentrations and/or binding affinity for T. To date, 71 genetic variants of albumin have been identified. One of these albumin variants, Roma c.1033G > A, has been linked to reduced binding of T [20]. Lower binding affinity for albumin, which has also been demonstrated for all other steroid and thyroid hormones, has raised discussion about the relevance of this albumin-bound fraction. Indeed, the albumin-bound fraction could, because of more probable spontaneous dissociation, potentially also have an effect on biological activity [21, 22]. Hence, the sum of the free and the albumin-bound fraction was determined to constitute the biologically available (or bioavailable) amount in circulation (Fig. 1). More recently, however, the interaction between the albumin-bound and free fraction has become even more complex. The binding dynamics of T to albumin have been generally believed to be in a 1:1 stoichiometric way. However, the structural location of the binding sites in albumin by two-dimensional nuclear magnetic resonance has shown that the carrier protein possesses at least three binding sites for T, each with its own association constant. Moreover, evidence has been provided that these binding sites are allosterically coupled and, importantly, shared with free fatty acids and some commonly used drugs, such as naproxen, warfarin, and exogenous glucocorticoids [23, 24]. Binding site competition and allosteric coupling can in certain conditions, especially postprandial and in circumstances of heightened levels of fatty acids and drug usage, result in displacement of T from its binding site thereby potentially affecting T bioavailability and/or metabolism [25, 26].
The link between binding proteins and the free hormone hypothesis
All BPs, whether specific or non-specific, together control tissue availability and metabolic clearance rate of a given hormone through regulation of the free hormone fraction. In the free hormone hypothesis, it is mostly this free fraction, and by extension the free hormone concentration, that accounts for the biological activity of hormone action
Unraveling the free hormone hypothesis: a model‑based approach
The free hormone hypothesis asserts that the biological activity of a given hormone is affected by the unbound (free) rather than the protein-bound plasma concentration [27]. In 1952, Recant and Riggs observed that patients suffering from nephrotic syndrome were euthyroid, without clinical signs of hypothyroidism, while in contrast, their serum levels of thyroid hormone were far below the normal range. They, therefore, hypothesized the existence of an, at that moment analytically undetectable, unbound fraction exerting biological activity rather than the protein-bound fraction. While suffering from hypoproteinemia and concordant low protein-bound thyroxine, patients would thus still have a sufficient supply of free, unbound, thyroxine [28]. This observation was later formalized by Robbins and Rall in 1957, stating that the concentration of free thyroid hormone in the blood is the main determinant of thyroid hormone action. In accordance, the amount of thyroxine metabolized may also be a function of the level of free thyroxine in serum [29]. In the following years, major advances were made as BPs were characterized and knowledge of a free hormone pool, which is in continuous equilibrium with the protein-bound pool, became well established [30]. Additionally, similar experiments on the sex steroids T and estradiol were conducted by Heyns and De Moor. They succeeded to isolate the free from the protein-bound fraction, thereby enabling them to study the binding kinetics of these sex steroids and their respective BPs [31].
Although many insights were achieved in terms of free serum hormones, it was not until 1989 that a sound mathematical and physiological model for the hypothesis was introduced by Mendel, referring to the hypothesis now generally accepted as the free hormone hypothesis [27]. Despite being broadly dispersed and acknowledged, it is commonly confounded with the assumption that only the non-protein-bound (free) fraction of hormones are able to cross the cell membrane to the intracellular compartment, referred to as the free hormone transport hypothesis [32]. While the free hormone transport hypothesis might be valid, it does not then automatically follow that only the free hormone concentration in the plasma can affect intracellular hormone concentrations and, therefore, exert biological activity. Indeed, this last statement was illustrated by Mendel’s mathematical models [27]. These models characterize the process of cellular steroid hormone uptake through four parameters: plasma flow rate, hormone dissociation rate from its BP, tissue influx rate, and intracellular elimination rate. In each of the proposed models, all representing different tissue types, one of these four factors operates as the rate-limiting factor in the uptake process. This in turn affects the validity of the free hormone hypothesis (Fig. 2). For instance, whenever intracellular elimination is considered the rate-limiting step in the net tissue uptake, the free hormone hypothesis is valid. This implies that intracellular hormone concentration, and, therefore, the biological activity of this hormone, is predominantly determined by its free fraction. The hypothesis is, however, invalid when flow or dissociation from the BP is rate-limiting. In the case of rate-limitation by the influx, the free hormone hypothesis may be either valid (influx via the free hormone pool) or invalid (influx via the protein-bound hormone pool). Providing this knowledge, some general observations can be stated. If a particular hormone exerts an intracellular effect, but its structure is not metabolically altered by the cell, uptake of that hormone is by definition elimination-limited, pleading a valid hypothesis. In contrast, tissues that rapidly metabolize and thus alter the hormonal structure, for instance, metabolic clearance by hepatic tissue, are not elimination-limited pleading against the hypothesis. In summary, these findings highlight that the free hormone hypothesis is not expected to be valid under all possible sets of conditions. A single steroid hormone can be subjected to different models depending on the tissue type [27].
The megalin/cubilin model does not impair the free hormone hypothesis
Although well established by Mendel, various researchers challenging the free hormone hypothesis have tried to demonstrate that the biological activity is mainly exerted by the protein-bound rather than the free fraction. The proposed mechanism for uptake of the hormone–BP complex is through the action of megalin and cubilin, via the process of receptor-mediated endocytosis. Megalin and cubilin are both large, multiligand, endocytic-membrane glycoproteins that are expressed in a wide variety of tissues, including but not limited to the kidneys, brain, endocrine glands, and gonads. Functioning either individually or as a cooperative unit, they are imperative for the endocytic uptake of low molecular weight molecules such as DBP, hormones, enzymes, and lipoproteins [33]. A megalin-knockout mouse model was used to provide evidence for the existence of a megalin-mediated cellular uptake pathway for the internalization of the T-SHBG complex. When megalin was absent, mice would exhibit an impaired testicular descent into the scrotum, delivering evidence that the uptake of the protein-bound fraction is also relevant for intracellular hormone action [34]. This research has, however, been challenged because of multiple flaws in the research design, hence not supporting the “bound steroid” hypothesis [35]. One of the counterarguments stated that mice lacking megalin suffer from severe vitamin D deficiency, accounting for the impaired gonadal development and function, rendering deductions for the T-SHBG complex incorrect [35].
While not applicable to T and SHBG, megalin- and cubilin-mediated endocytosis is, however, relevant in vitamin D metabolism. Being mainly expressed on the luminal brush border membrane of the renal proximal convoluted tubule, megalin and cubilin bind and reabsorb the vitamin D–DBP complex from the glomerular ultrafltrate, hereby preventing uncontrolled loss of vitamin D metabolites in urine. Additionally, megalin-knockout mice as well as patients with diabetes or chronic kidney disease (CKD), in whom renal megalin expression and function are reduced, show extensive loss of both vitamin D metabolites and DBP in the urine leading to a higher risk of vitamin D insufficiency [36, 37]. It is, however, important to state that the kidney is one of the major metabolizer organs in vitamin D biotransformation. Hydroxylation of 25(OH)D to its most active form, 1,25(OH)2D, by CYP27B1 is performed in renal tissue with the supply of 25(OH)D depending on megalin-/cubilin-mediated endocytosis [38]. Mendel’s mathematical model for the free hormone hypothesis describes that metabolizer organs or cells, in this case, cells of the proximal convoluted tubule, have by definition a non-elimination limited uptake and, therefore, are not subject to the free hormone hypothesis. Hence, megalin-/cubilin-mediated uptake of the vitamin D–DBP complex is not a valid counterargument to the free hormone hypothesis [27]. To summarize, while of crucial importance in vitamin D physiology, the action of the megalin and cubilin complex is not applicable to sex steroids and SHBG (Fig. 3).
Core support to the free hormone hypothesis: in vitro and in vivo data
Since its postulation by Mendel in 1989, the understanding of the free hormone hypothesis has advanced significantly. New insights could possibly contribute to the expertise of the daily management of many endocrine disorders. The clinical relevance and utility of this concept are determined by the relationship between the different fractions of the plasma hormone pool and the clinical manifestation of the corresponding affected endocrine axis. Currently, serum total T concentration is still most often used as the primary determinant for the assessment of androgen status in clinical practice. However, the consensus in the discussion on the most clinically useful measurement of sex steroids, total or free concentration, is yet to be reached [39]. In what follows, we present in vitro and in vivo data, delivering arguments in favor of the free hormone hypothesis, emphasizing the importance of SHBG and the relevance of the determination of the non-protein-bound fraction. Hence, this review pitches the measurement of free T as an added value on top of total T.
In vitro
Assessment of in vitro androgen bioactivity was studied by our research group using a stable HEK-293 cell line transfected with a human androgen receptor cDNA construct [40]. Expressing the human androgen receptor, androgen activity is reported through a luciferase-coupled androgen response element (Fig. 4). Using this reporter assay, it was demonstrated that adding recombinant human SHBG to SHBG-deficient serum significantly reduces androgen bioactivity in vitro, hereby confirming the inhibitory effect of SHBG on androgen exposure [41]. In an extension of this first experiment, the impact of genetic variants of SHBG on androgen bioactivity was also investigated in vitro. As mentioned before, SHBG genetic variants affect SHBG and total T concentrations. However, genetic variation in SHBG also alters the binding affinity constant for T and DHT [42]. According to the free hormone hypothesis, it should then follow that an alteration in binding affinity influences the equilibrium between the SHBG-bound and free fraction and thus ultimately impacts androgen activity. Indeed, the usage of genetic SHBG variants in combination with the luciferase androgen reporter assay modifies in vitro androgen bioactivity and reveals increased activity for genetic variants that lower binding affinity [41]. These results are in line with previously conducted in vitro studies that already demonstrated the inhibitory effect of SHBG on T action or uptake [43, 44]. Furthermore, we confirmed these findings in primary cultures of seminal vesicles [45]. In conclusion, these in vitro experiments highlight the importance of SHBG and its inhibitory effect on androgen bioactivity, conveying a major role to the carrier protein and further fortifying the validity of the free hormone hypothesis.
In vivo
Although rodents express an SHBG homolog locally in the testis, the androgen-binding protein, hepatic expression of SHBG, and SHBG secretion into circulation are absent postnatally. This deficit in circulating SHBG explains low levels and susceptibility to the fluctuation of T in rodents [46]. As such, to study the regulation of SHBG expression in vivo, a mouse model expressing a human SHBG transgene has been developed [47]. These transgenic animal models expressing human SHBG had higher concentrations of T compared to wild-type mice [47]. In more recent work from our research group, SHBG concentration in SHBG transgenic mice has been shown to increase with age and is remarkedly higher in males. In addition, total serum T and DHT are increased approximately 200-fold as opposed to wildtype and multiple T precursors (e.g., pregnenolone, androstenedione) were also found to be increased [45]. Curiously, although androgen levels were raised in SHBG transgenic mice, free T concentrations, measured directly by liquid chromatography with tandem mass spectrometry (LC-MS/MS) following equilibrium dialysis (ED), were not different compared to controls. However, despite similar free T levels in SHBG transgenic mice, luteinizing hormone (LH) levels were significantly higher than in controls. Moreover, following orchiectomy and implantation of a continuous-release T implant, free T was lower in transgenic compared to wild-type mice [45]. With these findings, we provided evidence that the rise in LH was explained by an SHBG-mediated decrease in free T, thereby triggering hypothalamic-pituitary feedback. The activated axis in its turn regulates and again raises free T concentrations by increasing testicular T production. Ultimately, free T and not total T is apparently the biologically active signal which directly feedbacks the hypothalamic-pituitary axis.
Phenotyping of the SHBG transgenic mice identified a partially compensated hypogonadism reflected in an increased LH together with a minor, but significant, weight reduction of the seminal vesicles and pelvic bulbus cavernosus and levator ani muscles. Despite an extremely high amount of total T in these animals, weight reduction of the most androgen-sensitive organs provides in vivo evidence of androgen deficiency attributed to the inhibitory effects of high levels of SHBG [45]. More profound, administration of anabolic doses of T or DHT led to seminal vesicle hypertrophy in wildtype mice that is, however, prevented by SHBG in transgenic mice. Next to the androgen-suppressing effect of SHBG, injection of castrated mice with T, DHT, or mibolerone (a synthetic androgen with negligible binding affinity for SHBG) revealed that SHBG prolonged the circulating half-life of T and DHT, but not of mibolerone [45]. This confirms that SHBG has the capacity to bind and lengthen the half-life of its ligands.
In summary, our group contributed a considerable amount of data from in vitro and in vivo experiments that lend direct and convincing support to the core statements that make up the free hormone hypothesis. First of all, the ability of SHBG to suppress androgen bioactivity was confirmed in both in vitro and in vivo studies. Second, this suppression is advocated by high-affinity binding between SHBG and T, thereby rendering the SHBG-T complex biologically inert. Free T on the other hand appears to be able to cross the cell membrane and carry out its androgenic biological effect. Finally, regulation of this non-SHBG-bound fraction is under the tight control of a negative feedback loop through the hypothalamic-pituitary axis. These arguments underline the physiological importance of SHBG and brand it as the gatekeeper of intracellular concentrations of T and its biological action in target tissues.
Absence of binding proteins in human subjects favors biological activity through free hormones
Apart from in vitro and in vivo data, compelling human data in support of the free hormone hypothesis is also available. As discussed above, BPs are intrinsically important in establishing and maintaining the equilibrium between the protein and the non-protein-bound fraction. In fact, BPs themselves bear evidence in favor of the free hormone hypothesis. A family with a missense mutation within the SHBG gene was identified, which resulted in unmeasurably low SHBG concentrations in plasma. SHBG-deficient serum was obtained from a male subject in this family who suffered from low libido and erectile dysfunction, symptoms related to decreased T. This person, however, had normal male gonadal and pubertal development as well as reproductive function. Despite his extremely low total T, the biochemical analysis uncovered normal free T [48]. The absence of elevated LH in this individual indicated a normal pituitary perception of T availability. The subject's symptoms (e.g., low libido and erectile dysfunction), possibly due to late-onset hypogonadism, could in this case not be completely explained by T deficiency alone, since free T was normal. An analogous finding recently emerged for DBP and vitamin D. A patient was found to have a novel pathogenic variant of the GC-gene, encoding for DBP, resulting in undetectable levels of DBP but without any clinical signs of vitamin D deficiency. Biochemical evaluation of vitamin D metabolites indicated lowered values of total 25-hydroxyvitamin D (25(OH)D) and 1,25-dihydroxyvitamin D (1,25(OH)2D), but normal range values of the corresponding free fractions of these metabolites [49]. Both clinical cases provide support for the free hormone hypothesis and deliver additional arguments for the relevance of free metabolites as preferred indicators for clinical manifestations of hormone deficiencies.
The impact of variability in SHBG on free hormones
*Life cycle evolution of SHBG levels
As mentioned previously, free hormone concentration not only depends on the binding affinity of a given hormone for its carrier proteins but also on BP concentrations. SHBG levels change during different stages of human life [50]. SHBG can be measured in fetal blood and amniotic fluid from 13 to 20 weeks of gestation and does not significantly differ at term (36–37 weeks) [51]. Human SHBG levels do not differ between male and female fetuses [52, 53]. Maternal SHBG levels on the other hand are subject to a significant five- to tenfold increase from early to mid and late pregnancy [54]. This gain in SHBG might serve as a mechanism to protect the mother against the pregnancy-induced increase of androgens [13]. This assumption seems indeed confirmed by a rare observation of a woman with extremely low SHBG, caused by a combination of two rare single nucleotide polymorphisms (SNPs), who exhibited symptoms of hyperandrogenism during pregnancy, whereas the twin daughters she gave birth to did not show any signs of virilization [55].
In neonates, SHBG increases shortly after birth, attributed to a parallel postnatal increase in thyroid hormones [56, 57]. This increase persists and SHBG remains high throughout childhood. During puberty, the concentration of SHBG falls in both sexes, with a stronger twofold decrease in men, resulting in adult men having only half of the SHBG concentration as compared to women of the same age [58, 59]. The reason for this remarkable decline during puberty remains poorly understood. In the last decade, however, several factors have been associated with this decline in SHBG. In a large cross-sectional study of 903 healthy subjects aged 6 to 20 years, body mass index (BMI), T, estradiol, and DHEAS were found to be negatively correlated with the evolution of SHBG levels from prepuberty into adolescence in men. In women, however, this negative association with SHBG was only observed for BMI and DHEAS [60].
In contrast to declining SHBG levels throughout male puberty, androgen concentrations increase. However, in adult and elderly men, the opposite occurs: SHBG concentrations increase whereas androgen levels decline. Indeed, the European Male Aging Study (EMAS) showed different cross-sectional age trends concerning SHBG and androgen status in community-dwelling men aged 40–79 years [61]. While SHBG is significantly higher in older age groups, total T and free T both display a decreasing trend with older age. This negative trend is, however, markedly stronger for free T, with a decrease of 1.3% per annum as compared to a decrease of only 0.4% per year for total T. These observations of decreased androgen levels and increased SHBG have been confirmed in the longitudinal Siblos Extension Study (SIBEX) [62]. In conclusion, age is an important contributor to variance in total T, free T as well as SHBG [61, 62].
*Genetic variability in SHBG influences hormonal status
Production of SHBG is a complex process that is influenced by hormonal, nutritional, metabolic, and pharmacologic factors [63, 64]. Additionally, interindividual variation in SHBG levels has been shown to be highly heritable (between 30 and 80%). SNPs are important coding and/or regulatory sequence modifications in the SHBG gene that alter transcription, translation, and thus the production of SHBG in general. These SNPs can explain the relationship between genetic background and interindividual variation of SHBG and by extent, sex steroid status. Various polymorphisms in the SHBG locus and the X chromosome have been linked to substantial variations in SHBG and/or T concentrations (Table 2). Analysis of genome-wide association data of more than 8000 men identified that two SNPs within the SHBG locus are independently associated with serum T concentrations. Rs12150660, located 11.5 kb upstream of the major transcription start site of SHBG, represented the SNP with the strongest genotype-dependent difference in T. Mean serum T concentrations are found to be highest in men with the TT genotype, lowest for the GG genotype and situated in between for the GT genotype of this SNP. For the second SNP, rs6258, a rare missense polymorphism located in exon 4 of SHBG, lower concentrations of T were observed for the CT vs CC genotype. Additionally, as rs12150660 and rs6258 are located within the SHBG locus, a relevant association with SHBG concentration was demonstrated for both SNPs [65–67]. Differences in serum T concentrations for both SNPs, however, were still significant after adjusting for these altered SHBG concentrations [65]. Furthermore, the CT genotype for the rs6258 polymorphism results in reduced binding affinity of SHBG for testosterone. In fact, because of the lower binding affinity of SHBG, the percentage of free T was 22% higher in individuals with the CT vs the CC genotype [65]. For a third SNP in the SHBG locus, rs727428, the T allele accounted for a decrease in both SHBG and T levels, without affecting calculated free T [66, 68].
Important genetic polymorphisms have also been identified in the promotor region of SHBG, adjusting its expression levels. First, total T, and not free T, was elevated in subjects with six repeats, as compared to seven or more, of the (TAAAA)n repeat polymorphism. This effect occurred independently from SHBG levels [69]. Other studies confirmed this observation, although their data also suggest an increase of free T in subjects with nine or more repeats [70, 71]. Furthermore, the number of (TAAAA)n repeats was also inversely related to SHBG levels [69, 70]. Second, for the rare homozygous AA genotype of rs1799941, an increase in both serum SHBG and T was observed as opposed to the reference genotype [66, 69, 72, 73]. Although more recently the same AA genotype of rs1799941 was linked to lower calculated free T [74], this was not preceded by findings in earlier studies [66, 69, 73]. Finally, for carriers of two other SNPs, rs6257 and rs6259, a decrease and, respectively, an increase of SHBG was observed [75].
Similar to the polymorphisms specified above, all located on chromosome 17 within the SHBG gene or its promotor, associations to serum androgen levels have been reported for loci on other chromosomes. Rs5934505 is an SNP located at Xp22, positioned 79 kb downstream and 145 kb upstream of, respectively, FAM9B and FAM9A (family with sequence similarity 9, member B and A), two genes that are expressed exclusively in the testis [76]. FAM9A and FAM9B, are believed to be involved in, among other, the formation of the synaptonemal complex and DNA replication/recombination during cell division. Nonetheless, the physiological importance of these genes remains largely unclear [76]. In men presenting with a T versus C allele of rs5934505, a decrease in total and calculated free T, but not in SHBG concentrations was observed [65, 77]. Another SNP, rs10822186 at 10q21, has also been associated with changes in T levels in men. This locus was connected to JMJD1C, a gene that might be involved in testosterone synthesis. A genetic variation in this gene may cause a change in circulating androgen levels [77].
Analogous to SHBG, comparable experiments have been carried out for DBP. As the major carrier for vitamin D in circulation, it serves a primordial role in determining total and free concentrations of vitamin D [3]. Similar to SHBG, over 120 genetic variants of DBP have been discovered of which three major polymorphic alleles are the most relevant ones (GC1f, GC1s, and GC2), yielding six allelic combinations and three corresponding phenotypes [78]. A variation in geographical distribution is seen in these phenotypes, as for instance in Africans, GC1f is the most common allele while in Europeans GC1s is most frequently found [79]. This genetic variation has been deemed the main argument for the observation of lower levels of both DBP and 25(OH)D in subjects of African versus European origin, while levels of bioavailable 25(OH)D were similar in both ethnic groups [80]. However, as no racial difference in DBP was observed when using a polyclonal assay or applying LC-MS/MS, this conclusion was most likely the result of a monoclonal assay bias, confirming poor vitamin D status in subjects of African origin [81, 82].
Regulation of SHBG levels
Biosynthesis of SHBG is mainly situated in hepatocytes and transcriptional control of the SHBG gene is, as discussed in the previous section, influenced by various factors [83, 84]. A mouse model with a gene addition of the 4.3 kb human SHBG uncovered that upstream of exon 1, a sequence of approximately 0.9 kb suffices for high gene expression levels [47]. Further characterization of this upstream region identified binding sites for chicken ovalbumin upstream promoter transcription factor (COUP-TF) and hepatocyte nuclear factor-4 alpha (HNF-4α) [85]. Competing for a mutual binding site, HNF-4α and COUP-TF create a functional antagonism, respectively, actively recruiting or blocking the transcriptional machinery, which is essential for the expression of SHBG in the liver. Their interaction serves as a molecular ‘on-off switch’ mechanism that explains the acute response of SHBG expression to changes in metabolic states [85].
Levels of SHBG in circulation are governed and affected by several determinants, summarized in Table 3. A consistent finding is a negative association between SHBG and BMI [86, 87]. Other indices used in the assessment of central obesity, such as waist circumference and waist-hip ratio, also exhibit this negative association with SHBG [88]. Indeed, male and female subjects suffering from obesity show lower levels of SHBG compared to their non-obese peers [87, 89]. In fact, a negative association between obesity and SHBG is even present in overweight and obese children [90]. Moreover, following bariatric surgery, SHBG levels rapidly increase [91, 92]. Furthermore, this relationship between plasma SHBG levels and obesity is stronger than the positive association of SHBG with age described earlier. SHBG concentrations are highest in non-obese older men and lowest in obese younger men [87]. In contrast, in female patients with anorexia nervosa, SHBG concentrations were lower compared to normal weight subjects and increased to the normal range after adequate weight gain, thus being a reliable index of nutritional status [93]. Apart from BMI, other conditions influence SHBG levels. For instance, in both sexes, HIV-positive individuals have increased SHBG concentrations compared to their seronegative peers [94–96]. Thyrotoxicosis is also featured by an increase in SHBG as well as increased total T concentrations [97]. Hypothyroidism on the other hand is accompanied by a decrease in both SHBG and total T [98], which is reversible with adequate treatment [99].
Fluctuation of SHBG can also arise from the administration of exogenous substances that display estrogenic and/or androgenic properties. Among others, estrogen-containing products, like combined oral contraceptives (COC) but not their progesterone-only counterparts, are associated with an increase in SHBG concentration and other major changes in several plasma protein profiles [100]. Tamoxifen, mainly used in the treatment of breast cancer predominantly displays an estrogen-like effect on hepatic tissue, stimulating SHBG production [101, 102]. Similarly, the use of mitotane in the treatment of adrenal carcinoma is associated with an increase in plasma SHBG levels [103]. Contrary to drugs with estrogenic effects, danazol, mainly used in the context of endometriosis, is a compound of androgenic nature. Binding to SHBG with high affinity, it is capable of altering the binding affinity of SHBG for T thereby displacing it from its carrier, subsequently increasing free T, and eventually leading to decreased concentrations in SHBG [104–106]. Affecting sex steroid balance in a different way, drugs without innate estrogenic and/or androgenic properties also exert their effect on SHBG levels. Administration of some antiepileptic drugs (e.g., phenobarbital, phenytoin, and carbamazepine) can induce hepatic microsomal enzymes. This results in an increase in SHBG concentration that in turn influences free circulating androgen levels, lowering T bioavailability [107–109]. Administration of statins is associated with a decrease in SHBG [110, 111]. In another example, treatment with synthetic glucocorticoids, for instance, dexamethasone, has also led to decreased SHBG levels in women with PCOS and in children with acute lymphoblastic leukemia [112, 113]. Clearly, SHBG levels in circulation respond to different endocrine and metabolic signals. This in turn affects circulating T levels and hence designates SHBG as a potential biomarker for the disease.
SHBG: a potential biomarker of disease?
In the last decade, SHBG has been the center of attention in research on type 2 diabetes mellitus (T2D), obesity, PCOS, male hypogonadism, liver disease, bone health, and even cancer. All these disorders have been associated with altered SHBG levels [114]. As a result, the clinical usefulness of SHBG and its potential as a biomarker of disease has been questioned. To assess this potential, the classical definition of a biomarker is of great importance: “A defining characteristic that is measured as an indicator of normal biological processes, pathogenic processes or responses to an exposure or intervention [115].” Additionally, to be able to classify SHBG as an ideal marker, among others it should be specific to a particular disease and able to differentiate between different physiological states [116]. For the following reasons, SHBG fails to meet this qualification. First, almost all SHBG associations result from cross-sectional study designs. As no prospective studies have been conducted, statements on causation are lacking. Second, SHBG shows a strong correlation to T, making it difficult to observe an independent SHBG effect. Finally, and vice versa, designing a study to reveal an independent effect of SHBG proves to be challenging, as SHBG and T are tightly bound. In summary, while SHBG may not classify as an ideal diagnostic biomarker, it shows associations with different disorders. As such, and for further research purposes, we deem it useful to provide a concise overview of these associations.
*Glycemic control and progression to metabolic syndrome
SHBG has been implicated as a contributing factor to T2D and insulin resistance (IR), entities closely related to obesity
*All previous data show a convincing association between obesity/BMI, T2D, METS, and SHBG. Focusing on metabolic disturbances, the inclusion of SHBG in diagnostic evaluation protocols could be an asset.
*SHBG in nonalcoholic fatty liver disease
Nonalcoholic fatty liver disease (NAFLD) is a common cause of chronic liver disease, possibly progressing to cirrhosis or hepatocellular carcinoma. NAFLD is mainly characterized by the accumulation of triglycerides and fatty acids in the liver, in the absence of a secondary cause of fatty liver such as excessive alcohol use or viral hepatitis.
*While the link between SHBG and NAFLD progression is not yet fully elucidated, SHBG clearly shows associations with NAFLD.
*SHBG and bone health
A link between SHBG levels and osteoporosis has also been reported. [145].
*All of these studies, both in men and in women, suggest an association between SHBG, bone health, and fracture risk.
Diagnosing hypogonadism in men: total versus free testosterone
Male hypogonadism is a clinical condition that can be subdivided into two types. Organic hypogonadism is characterized by a structural, congenital, or destructive disturbance of the hypothalamic-pituitary-gonadal (HPG) axis, leading to the inability of the gonads to produce a sufficient amount of androgens resulting in clinical signs of androgen deficiency. Functional hypogonadism on the other hand refers to identical clinical signs and coexisting androgen deficiency without an intrinsic pathology of the HPG axis [165]. These androgens are, during the male life span, needed to promote masculine development/virilization and growth, and are of vital importance in reproductive functioning [166]. Manifesting differently between male individuals, symptoms of androgen deficiency vary according to the age of onset, the severity of T deficiency, androgen sensitivity, and previous and/or current use of T replacement therapy [166]. These symptoms cover a broad clinical spectrum (Table 4). It is, however, clear that a number of these complaints overlap with other chronic diseases such as obesity and diabetes mellitus. In fact, multiple studies confirmed the association between low or inappropriate T levels in men and T2D and obesity [167–170]. Furthermore, because of the high prevalence of cooccurrence of T2D and obesity, low T was unsurprisingly also associated with metabolic syndrome [171, 172]
While these clinical symptoms are the main motivation for patients to visit a physician, the diagnostic emphasis of androgen deficiency has heavily shifted onto biochemical measurement of circulating T. Confirmation of deficient gonadal production of T is, therefore, reflected in the measurement of low total T in serum [173]. Despite being the first-line test in the diagnosis of hypogonadism in all practice guidelines, there is no agreement between these guidelines concerning differentiating low from normal circulating T (Table 5). Although there are no issues concerning test sensitivity and picking up low-range concentrations of total T, a precise definition of the lower limit of ‘normal’ total T remains ambiguous. Multiple studies attempted to establish a reference range to achieve generally accepted values for total T in young men, ending in diverse intervals for all different cohorts [174–177]. A collaboration of European and American researchers and clinicians argued that these differences could be attributed to geographic region, interlaboratory assay validation as well as biological and environmental factors. In their effort to harmonize reference ranges, they established 264 to 916 ng/dL as a normal interval for total T in European and American non-obese men aged from 19 to 39 years. Using a reference method from the Centers for Disease Control and Prevention (CDC) they concluded that previous cohort inter-variability was mainly due to a substantial amount of inter-assay variation [178]. The use of this reference method should allow for interlaboratory harmonization of total T reference ranges in men. Caution, however, is still advocated when classifying men according to total T values. Currently, obesity is one of the leading major health problems, with over 650 million people affected worldwide [179]. Imposed by their obese status, these men are prone to lower total T as well as lower SHBG concentrations, implying a substantial risk for misclassification when using ‘normal’ reference ranges [180, 181].
While we agree that the measurement of total T is and should remain the first step in diagnosing male hypogonadism, the results of an observational study conducted by our research group confirmed the potential added value of free T in this context [182]. In our study, despite men having normal total T levels, a low calculated free T serum level was associated with androgen deficiency-related symptoms (sexual, physical, and psychological functioning, biochemical measurements, body composition, and bone measurements). Moreover, cases of low total T in combination with normal calculated free T were not associated with hypogonadal signs. With regard to our results, we recommend that free T should be included on top of total T in the evaluation of hypogonadism in men, especially in obese subjects [182]. Moreover, in HIV-positive men, underdiagnosis of male hypogonadism would have occurred in one-third to half of the study subjects if only total T was measured as opposed to combining it with free T [183, 184]. These data underline the importance of free T values and adding this parameter to biochemical testing could, therefore, increase the diagnostic sensitivity of male hypogonadism. Using this knowledge, the Endocrine Society (ES) decided to amend its guidelines on the diagnosis of hypogonadism in men, including free T as a novel parameter [7]. In the diagnostic approach proposed by the ES, total T should be measured in a fasting subject on two separate mornings. Additionally, determining free T is either advised when men are subject to conditions that alter SHBG levels or when the initial measurement of total T was below or close to the lower limit of the normal range. Furthermore, recommendations on determining free T are either direct measurement by ED or through a calculation using total T, SHBG, and albumin values [7].
Free sex steroids in women with polycystic ovarian syndrome
PCOS is one of the most common endocrine disorders worldwide, with, depending on the criteria used, a prevalence rate of 4% up to 18% [185]. PCOS leads to infertility, menstrual problems, and clinical manifestations of hyperandrogenism in women of childbearing age [186]. Women with PCOS are often obese, develop IR, and are at risk of developing T2D [187, 188]. The pathological mechanism by which PCOS arises is very complex, and multifactorial, and still remains largely unclear, but appears to be a disbalance in hormones, androgens, and insulin in particular [189]. Furthermore, obesity may exacerbate features of PCOS by worsening IR and stimulating hyperandrogenism [190, 191].
In the diagnostic workup of PCOS, androgen excess is usually assessed by measurement of total T [187]. In routine clinical practice, total T is most frequently measured by immunoassay methods. However, some caution is warranted as commercially available immunoassays may not be suited to accurately and precisely determine low T concentrations in women [192]. As a consequence, total T by direct immunoassay misclassifies PCOS in 1 out of 3 subjects [193]. Hence, the acquisition of total T results in women should be done by chromatography-mass spectrometry, which has become the gold standard for the measurement of sex steroids [39, 187]. Notwithstanding more accurate total T results can be achieved with LC-MS/MS, total T may be an insensitive marker for hyperandrogenism in women [193]. In contrast to total T, free T seems to be a better marker to determine hyperandrogenemia in women with PCOS [187], provided that an accurate and high-quality methodology is used for measured or calculated free T [193, 194].
Together with free T, SHBG has recently been recognized as a useful diagnostic marker and therapeutic target for managing women with PCOS [195]. A meta-analysis showed that subjects with PCOS had remarkably lower levels of SHBG and that this effect was even more pronounced in subjects with concomitant obesity [196]. In the evaluation of androgen excess in women suspected of PCOS, it is thus important to evaluate both free T and SHBG, as they reflect both metabolic and ovarian disturbances associated with this disease [197].
Methodology on calculated and measured free testosterone
Provided the evidence that free T gains clinical importance in the diagnostic landscape of male hypogonadism, several methods for the assessment of this free fraction have been developed and are currently available. Moreover, not only can free T be of value in the clinical context of hypogonadism but, as discussed in another section of this review, due to alterations in SHBG and total T, free T might be of interest in several other pathologies. In the clinical setting, assessment of free T can be carried out either by direct measurement or through calculation.
Measurement of free testosterone
Measurement of serum-free T through ED is, as recommended by ‘The Princeton Consensus Statement’ and many other publications, the well-established reference method [198]. This technique allows for dialyzing a patient’s serum or plasma across a semipermeable membrane, retaining protein-bound T in the process. Whereas SHBG and albumin-bound T are withheld from the dialysate, unbound (free) T can freely pass. After reaching equilibrium, the dialysate can subsequently either be analyzed by a direct or indirect method, thereby describing the technique as direct or indirect ED. In direct ED, measurement of the free hormone concentration in the dialysate is directly performed by LC-MS/MS. Indirect ED on the other hand uses a labeled (usually tritium) tracer steroid, and the measured total steroid concentration, to proportionally determine the free steroid concentration. Indirect ED, as compared to direct ED, is subject to several limitations, such as tracer instability, tracer purity, and the need for a dilution step, that all introduce possible sources of added total error to the free steroid measurement [199, 200]. Hence, direct ED using LC-MS/MS is the preferred method for the measurement of free serum hormones.
Different other methodologies for assaying free fraction steroids exist, but these are beyond the scope of this review. Briefly, LC-MS/MS coupled ultrafiltration (UF) constitutes a comparable alternative to ED. UF has also previously been described in the validation of a reference measurement procedure for free T [200]. Little data exist on the validation of UF versus ED, yet UF is a relatively convenient technique and when properly validated can display equal performance to ED [199, 201, 202]. Similar to ED, UF deals with its own technical issues. The most common pitfalls of UF are due to non-specific binding, steroid adsorption to the filtration membrane, and protein leakage through the filtration membrane [200, 203]. Additionally, an analog-based immunoassay has been developed. These methods claim to measure free hormones directly in serum. Nevertheless, inadequate accuracy, bias, and dependency on T rather than free T are major drawbacks in these analog-based assays [204–206]. Comparative studies of this analog-based immunoassay and ED confirmed that this technique does not detect nor quantify free T and that it should, therefore, not be used as an assay for free T [205, 207, 208]. This led the ES to recommend against the application of this assay in clinical settings [39]. While the use of this analog-based technique is clearly decreasing, surprisingly, a survey conducted in Northern America acknowledged that in 2011 approximately 33% of queried clinical laboratories still used this analog-based test as the primary test to measure free T [209].
As mentioned before, it is not only possible to assess the free fraction, but also the bioavailable fraction of sex steroids. The bioavailable fraction of T consists of both the free T fraction and the albumin-bound T fraction. In this definition of bioavailable T, the albumin-bound fraction is designated part of the usable T pool because of the low Ka between T and albumin, making the binding between ligand and carrier prone to dissociation [21, 22]. The gold standard for measurement of bioavailable T is a direct precipitation method with either ammonium sulphate or concanavalin A. Both substances form a complex with SHBG, resulting in precipitation of the carrier and by extension, the SHBGbound T. Addition of a tracer element, 3 H-testosterone, to the sample or direct measurement by immunoassay or LC-MS/ MS, allows for assessment of both the free and albumin-bound fraction in the supernatant [208, 210]. However, this method is rarely used in diagnostic laboratories as it is not automated, is cumbersome to execute, and may involve radioactive tracer elements. Furthermore, because it is not standardized, large variability in bioavailable T results may exist between laboratories [208]. Additionally, the measurement or calculation of bioavailable T is not mentioned in any clinical guidelines [6, 7]. As such, bioavailable T is only used in a limited way and evidence of clinical associations is scarce. For now, we would not recommend using bioavailable T as an alternative to free T.
Calculation‑based free testosterone
Because of the limited availability of ED in clinical settings and the unsuitability of direct free testosterone assays, values for free T are frequently obtained through calculation. Multiple calculation algorithms, using other serum-measured biochemical variables, e.g., SHBG, albumin, and total T concentrations, have been computed. These formulas depend on the precision and accuracy of the methodologies of their included variables. Immunoassays for total T; SHBG and albumin are validated diagnostic techniques. Despite their high availability and improvements over the last 30 years, performance issues with regard to precision and accuracy still exist. On the other hand, LC-MS/MS provides a more accurate measuring method for total T [39, 211]. A wide spectrum of algorithms for free T exists, which can broadly be divided into three categories (Table 6). First, several equations were derived from the general law of mass action. Association constants for albumin and SHBG used in these formulas were obtained from in vitro experiments by linearizing data through Scatchard analysis [16, 208, 212]. A second category contests the principle of Scatchard analysis, refuting the assumption that SHBG expresses linear binding kinetics [213]. This model takes into account allosteric interaction between binding sites for T on the SHBG homodimer. Empirical models exploiting bootstrap regression methods on cohort datasets comprise the third and last category [214, 215]. All these models have respective strengths and intrinsic limitations, as described in an extensive review [5].
An alternative method for free fraction T calculation is the use of the free androgen index (FAI). The FAI is calculated by the ratio of total T to SHBG, delivering an alternative way of estimating free androgens. Because of its accessibility, the FAI is widely utilized in clinical laboratories and clinical studies spanning different disciplines in medicine [216, 217].
Additionally, in many research designs on hyperandrogenism in women, FAI is a key parameter of interest [218, 219]. Caution, however, should be exercised, as the FAI is highly impacted by SHBG concentrations. In human subjects, male or female, in whom SHBG concentrations are altered, FAI could increase or decrease dramatically. This results in an artificial over- or underestimation of free fraction androgens [220]. As disserted previously in this review, SHBG concentrations are subject to change in pathologies such as obesity, diabetes, and metabolic syndrome. It is, therefore, not advised to use FAI as a clinical decision-maker.
Agreement between measured and calculated free testosterone
Regrettably, all the methods described above leave researchers and clinicians with the critical question of which method provides the best fit to delimit free T values. To address the matter, a Belgian research consortium including some of the authors of this review, assayed over 300 serum samples of human males and females for free T [221]. Measurement of free T on all samples was performed by gold standard LC-MS/MS coupled ED. In addition, free T for each sample was calculated using the Vermeulen, Zakharov, and Ly/ Handelsman models. Median bias, expressed by the ratio of calculated to measured free T, was 1.19, 1.0, and 2.05 for the Vermeulen, Ly/Handelsman, and Zakharov models, respectively. Our data also demonstrated that both Ly/Handelsman and Zakharov methods do not operate independently of SHBG, total T, and to a lesser extent albumin values [221]. This is particularly important in distinct conditions where SHBG and total T are in the lower range, increasing the probability of underestimating free T by calculation. Underestimation may pose a problem, especially in clinical settings of hypogonadal men (low total T), obesity (low SHBG), and women with PCOS (low SHBG). Paradoxically, assessment of these patient groups is also commonly the most relevant reason for requesting free T. In contrast we concluded that, although overestimating free T by 20–30%, the formula according to Vermeulen was independent of SHBG, total T, and albumin [221]. Taken into consideration the moderate bias level, but most importantly less influenced by the latter three variables, the Vermeulen formula, for the time being, still appears to be the most robust approximation and deserves our recommendation as a free T calculator for clinical use.
Free testosterone in clinical practice: limitations and approaches
Direct measurement of free T using LC-MS/MS coupled ED is currently, as previously discussed, the gold standard. Essentially obtaining accurate results, execution of this assay is, however, very labor intensive and technically challenging, resulting in limited availability of the method in everyday clinical routine [222]. In fact, currently non-automated, the process is subject to operator-dependent errors. Apart from these technical difficulties, assay performance is also affected by various conditions and error sources, governing high assay variability. Adhesion of components to the membrane or the dialysis chamber, dilution, assay temperature, and buffer characteristics such as volume, pH, and composition all impose differential effects on binding parameters and equilibrium conditions leading to distinct quantifications [39, 200, 208, 223–226]. In addition to these technical issues, the deployment of an ED technique adds extra costs to clinical laboratory practice [227]. As such, LC-MS/MS coupled ED is mostly only available in academic laboratories. Additionally, the measurement of (free) T is of less commercial interest, unlike (free) thyroid hormones. Whereas thyroid diagnostics make up a large part of daily laboratory tests requested by almost all clinicians, T is mostly only requested by endocrinologists and urologists. Hence, in the past decades, more resources have been invested in clinical thyroid diagnostics, enabling the commercialization of kits for the clinical laboratory allowing direct measurement of free thyroid hormones. In contrast, fewer funds were, and still are, directed at the development of assays for free T.
There is, however, a fairly good agreement between measured and free T calculated by the Vermeulen formula, if validated against ED [221, 228]. In fact, in clinical settings where direct measurement of free T is not available, the Vermeulen formula can serve as an acceptable clinical alternative. In addition, calculation as opposed to direct measurement also presents as a more convenient and cheaper quantitative metric. Summarized in Table 7, users of free T calculators should on the other hand also consider the different imperfections of these clinical utilities. Most importantly, in these models, free T is calculated through values for SHBG, albumin, and total T. Consequently, calculation quality depends on the performance characteristics of the assays used to determine these three variables. Second, concerning the interaction between SHBG and T, variability and assumptions in terms of binding affinity, stoichiometry, and allosteric interaction produce different quantities of free T in different models [5, 208]. Third, all of these models are in fact simplified versions of the true binding milieu and may potentially not include all variables affecting the equilibrium between total and free T (e.g., the role of CBG). Finally, for both measured and calculated free T, reference ranges may not be universally valid. Depending on the method to assess free T as well as the characteristics of the cohort, different intervals may be generated [177, 229]. In extension, there is no consensus on a universal cut-off between low and normal values for free testosterone. Further research efforts on harmonizing these reference ranges are, therefore, needed.
*In summary, we would suggest a pragmatic and practical approach to assessing androgen status in a clinical setting. Measurement of total T and SHBG is standard of care, which in any case also allows for the additional calculation of free T. In an altered SHBG-binding milieu, or when total T is borderline normal or below the lower limit of normal, accurate determination of free T can subsequently be carried out using LC-MS/MS coupled ED
Linking clinical endpoints to free testosterone
*Androgen‑related clinical endpoints
Illustrated in the previous sections of this review, free T has its role in the diagnosis of male hypogonadism. In the assessment of the quality of life in hypogonadal patients, physicians mostly rely on androgen-dependent clinical endpoints. While these endpoints have been profoundly studied in terms of total T concentrations, evidence on the link between free T and these endpoints is more scarce. Interestingly, while demonstrated that, in the case of the Vermeulen formula, calculation of free T leads to a constitutive positive bias of 20–30% compared to direct measurement using ED, almost all current research uses one of the previously mentioned calculation models to study associations with clinical endpoints.
*Clinical outcomes in other diseases
In related research, calculated and/or measured free T, as opposed to total T, were found to correlate stronger with clinical outcomes of NAFLD, PCOS, and hypertension [143, 237, 238].
*Although free T has been clearly associated with several clinical endpoints of interest, the fact that these conclusions are based upon calculated, and not directly measured, free T is a less potent element of the free hormone hypothesis statement. As discussed before in this review, free T calculations have several limitations. However, repeating these clinical studies using direct ED measurement would impose technical and logistical challenges, as well as require a great deal of effort from all scientific collaborators. While, however, not available at this moment, endeavors are underway to optimize the implementation of free T in clinical practice.
The need for free testosterone in the clinical setting: why and when?
Men with established organic hypogonadism are usually treated with T replacement therapy. Available in various formulations, androgen replacement serves the goal of establishing and/or sustaining secondary sexual characteristics in these men. Detailed specifics on administration, intended and adverse effects of T replacement therapy are beyond the scope of this review and have been discussed elsewhere [166]. Prescription of T supplements has increased phenomenally during the past 2 decades, especially in the larger group of men suffering from functional hypogonadism [247]. Nevertheless, T supplementation is not without risk and could potentially be harmful concerning cardiovascular risk in the elderly [248]. Currently, the path to diagnosing hypogonadism is still directed towards biochemical dosing of total T. However, conditions that affect SHBG levels unequivocally affect total T concentrations. Free T on the other hand is not necessarily impacted by this change in SHBG. The question rises of how free T can be integrated into a clinical workflow concerning male hypogonadism. More systematic use of free T on top of total T could reduce overtreatment and T prescriptions.
*With these combined results of the cross-sectional and longitudinal analysis, we clearly highlight that the sole use of total T could lead to either under or overdiagnosis of hypogonadism. To avoid these pitfalls of misdiagnosis, in particular when total T is borderline low and/or SHBG concentration is altered, the addition of free T to the diagnostic landscape of male hypogonadism is of incontrovertible value.
Conclusion
This review delivers strong arguments from in vitro, in vivo, and human experiments in support of the free hormone hypothesis. While we also acknowledge particular shortcomings in the hypothesis, we believe free fraction hormones as described by the free hormone hypothesis provide clinical utility in patient healthcare. Assessing free T on top of total T as a standard biochemical parameter in the diagnostic workflow of male hypogonadism is clinically relevant when total T is borderline low and/or SHBG concentration is altered. Furthermore, the measurement of free T in PCOS and other endocrinological conditions can be of diagnostic value. As LC-MS/MS coupled ED is not routinely available, for now, calculated free T by means of the Vermeulen formula is preferential to assess andrological status in the clinical setting. However, several barriers remain in the implementation of free testosterone into routine clinical practice (Table 8). More in-depth research is needed on the interaction between steroid hormones and their BPs. Further exploration of the steroid binding milieu and development of corresponding models (e.g., reappraisal of free hormone calculators) can establish the clinical relevance of these free fraction hormones. In addition, efforts should be made to develop new or improve existing free hormone methodologies. Improving availability and accessibility to direct measurement methods are important milestones and will deliver added value to the diagnostic landscape of hormonal exposure in various endocrinological conditions.
2018
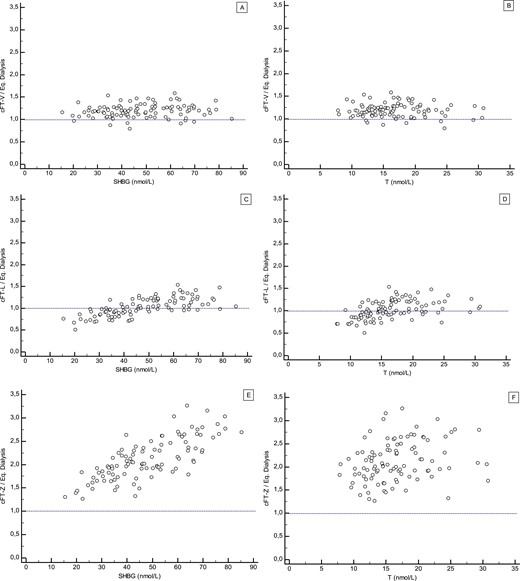
Reassessing Free-Testosterone Calculation by Liquid Chromatography–Tandem Mass Spectrometry Direct Equilibrium Dialysis
Reassessment of FT in women and men with state-of-the-art methodology confirms previously established FT percentages but highlights limitations of differen
